Pyrroloquinoline quinone attenuates cachexia-induced muscle atrophy via suppression of reactive oxygen species
Introduction
Cachexia, a wasting syndrome, is most commonly observed in the majority of patients with advanced cancer including lung cancer, liver cancer, gastric cancer, renal cancer, etc. (1-3).
The hallmark of cancer associated cachexia is muscle wasting (4). Cachexia is characterized by inexorable muscle atrophy that largely affects patient prognosis and contributes significantly to cancer morbidity and mortality (3,5). To date, effective countermeasures have been still deficiency to alleviate muscle wasting. Inflammatory mediators [tumor necrosis factor-α (TNF-α), interleukin-1 and interleukin-6] are key contributors to the development of cancer cachexia, which is the driving forces of skeletal muscle atrophy caused by cancer cachexia (6,7). TNF-α was often used to induce a model of muscle atrophy in cancer cachexia (8-10). Therefore, to elucidate the molecular mechanism and develop targeted therapies of cachexia associated muscle atrophy is of significant importance.
The balance between protein synthesis and degradation is crucial to preserve muscle healthy. Skeletal muscle atrophy occurs when protein degradation exceeds protein synthesis (11). Skeletal muscle atrophy is closely related to a reduced myofibrillar protein which results from both increased proteolysis and decreased protein synthesis (2). The ubiquitin-proteasome pathway (UPS) and the autophagy-lysosomal pathway (ALP) are two main intracellular proteolysis systems (12,13). The UPS is well recognized to be involved in mediating muscle atrophy, and is a key factor of muscle atrophy under various physio-pathological situations (14,15). muscle RING finger-1 (MuRF1) and muscle atrophy F-box (MAFbx) are two muscle-specific E3 ubiquitin ligases of the UPS (16). Our previous study has shown that MuRF1 and MAFbx displayed significant up-regulation during skeletal muscle atrophy under various pathophysiological conditions (17-20). Likewise, MuRF1 and MAFbx displayed an up-regulation in muscle atrophy caused by cancer cachexia and cerebral ischemia (16,21,22).
Reactive oxygen species (ROS) generation is at relatively low level in skeletal muscle under physiological conditions (23). While elevated ROS led to increased protein degradation through increasing the expression of key atrophic factors such as MuRF1 and MAFbx (24). Our previous study had also shown that the gene expression of the positive regulators for ROS production were up-regulated and the negative regulators for ROS production were down-regulated in denervation induced muscle atrophy (20). Although growing evidence has suggested that high levels of ROS might play a crucial role in muscle wasting, and effective targeted therapeutic strategies are still deficient for muscle atrophy. The therapeutic strategy of skeletal muscle wasting with natural compounds has recently received more and more attention. Pyrroloquinoline quinone (PQQ) is a naturally occurring redox cofactor, and it is known to protect cells from oxidative stress (25). Nonetheless, the effects of PQQ on skeletal muscle atrophy caused by cachexia have not yet been clearly addressed. Therefore, the aim of this study is to determine the protective effect of PQQ on muscle atrophy.
In this work, we first detected ROS production and analyzed whether ROS were central for cachexia-induced muscle atrophy. Furthermore, the effects of PQQ administration on cachexia-induced skeletal muscle atrophy were estimated. PQQ administration could resist muscle atrophy, as evidenced by increased muscle fiber cross-sectional area (CSA) or diameter of myotubes, together with increased myosin heavy chain (MHC) levels and decreased ROS, MAFbx and MuRF-1 levels.
Methods
Cell culture and treatments
Mouse skeletal muscle C2C12 cells were purchased from the ATCC (American Type Culture Collection), and were cultured in high-glucose DMEM (Dulbecco’s modified Eagle’s medium) containing with 10% fetal bovine serum (FBS) at 37 °C in a humidified atmosphere with 5% CO2. Mouse skeletal muscle C2C12 cells were differentiated in C2C12 differentiation medium containing DMEM, antibiotics and 2% horse serum (Invitrogen, Waltham, MA, USA) for 7 days (19). For antioxidant treatment, 10 ng/mL recombinant mouse TNF-α (R&D Systems, USA) was added and incubated with the differentiated C2C12 myotubes in the presence or absence of N-acetyl-L-cysteine (NAC) (5 mM) or PQQ (Sigma-Aldrich, 80 µM) for 24 hours. Finally, the C2C12 myotubes were examined by morphometric or biochemical assays.
Western blotting analysis
Western blotting analysis was carried out as described previously (26). For the protein extraction, C2C12 myotubes were homogenized in the radio immunoprecipitation assay buffer and the extraction was quantified with Bio-Rad Protein Assay Kit (Bio-Rad, Hercules, CA, USA). Then, total proteins were subjected to SDS-PAGE (polyacrylamide gel electrophoresis) and transferred onto polyvinylidene difluoride (PVDF) membranes (Millipore, USA). Following, the PVDF membranes were blocked with 5% nonfat dry milk diluted in Tris-buffer saline (TBS), and incubated with primary antibodies: mouse anti-MHC (1:3,000) (R&D Systems, USA), rabbit anti-MAFbx (1:3,000) (Abcam, USA), rabbit anti-MuRF-1 (1:3,000) and mouse anti-tubulin (1:3,000) (Santa Cruz, CA, USA) at 4 °C overnight. Next morning, the PVDF membranes were incubated with the appropriate secondary antibody. The immunoreactions were performed by enhanced chemiluminescence (Thermo Scientific, USA) and the intensity values were obtained for further normalization against the internal reference.
Myotube diameter measurement and quantification
The diameter of myotubes was analyzed by MHC staining. Briefly, C2C12 cells were seeded and differentiated on the glass coverslips. Then C2C12 myotubes were fixed and incubated with mouse anti-MHC (1:3,000) (R&D Systems, USA) for 1 hour. When the antibody incubation fluid was removed, the slips were incubated with affinity-purified Alexa Fluor dye-conjugated goat anti-mouse antibody (1:100) (Life Technologies, USA) for 30 min. The slips were photographed with the fluorescence microscopy. The diameter of myotubes was evaluated by the Image J software (27).
Determination of ROS
The ROS content is measured by using dichlorodihydrofluorescein diacetate (DCFH-DA) staining (Sigma-Aldrich, USA). DCFH-DA is widely used techniques for directly measuring the ROS content (28). When DCFH-DA is oxidized by ROS, it would be changed into the fluorescent compound dichlorofluorescein (DCF). Briefly, C2C12 myotubes were washed with PBS and fresh DMEM without phenol red, and incubated with 10 µM of ROS sensitive dye DCFH-DA for 30 min in the dark at room temperature. Then, the C2C12 myotubes stained with DCFH-DA were immediately analyzed, and the ROS content was assessed by the increase in the fluorescence intensity of DCF. The fluorescence intensity of DCF was measured at an excitation wavelength of 488 nm and an emission wavelength of 519 nm. Mean fluorescence intensities were obtained by histogram statistics.
Statistical analysis
All data were expressed as mean ± SEM from at least three independent experiments. Statistical methods including Student’s t-test and one-way ANOVA were used to analyze the differences between groups. All statistical analyses were performed with the SPSS statistical software version 17.0 (SPSS Inc. Chicago, IL, USA). P values <0.05 were considered statistically significant.
Results
ROS generation is enhanced in TNF-α induced C2C12 myotubes atrophy
The ROS content was measured by using DCFH-DA staining in C2C12 myotubes in the presence or absence of TNF-α treatment. Results showed that the relative fluorescence intensity in C2C12 myotubes exposed to TNF-α was higher than that in the C2C12 myotubes without TNF-α treatment (P<0.05) (Figure 1A), which indicated that the ROS generation was enhanced in TNF-α induced C2C12 myotubes atrophy, and also demonstrated that ROS generation might play a central role in TNF-α induced C2C12 myotubes atrophy.
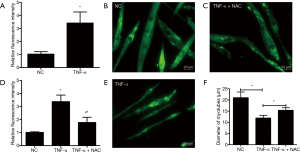
ROS are crucial for skeletal muscle atrophy induced by TNF-α
Next, the effect of increased ROS on the atrophic response induced by cachexia was determined by incubating myotubes with NAC, an antioxidant for ROS abolition, and assessing the diameter of myotubes. NAC treatments significantly relieved the decrease in the diameter of myotubes (Figure 1B,C,D,E) and the increase in ROS content (Figure 1F). Following, the influences of NAC on the MHC, E3-ubiquitin ligases MAFbx and MuRF-1 levels were examined by Western blotting. Treatments with 5 mM of NAC avoided the reduction of the expression of MHC, and also inhibited the increase of MAFbx and MuRF-1 levels (Figure 2). These results suggested that the increased ROS caused by TNF-α might play a critical role in muscle wasting.
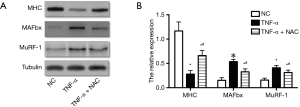
PQQ prevents ROS generation and muscle atrophy induced by TNF-α
In order to explore the effects of PQQ, a natural antioxidant, on the muscle atrophic effect induced by TNF-α, muscle atrophy and ROS generation were analyzed by incubating myotubes with PQQ, and evaluating the diameter of C2C12 myotubes and the ROS generation. The diameter of C2C12 myotubes was determined through MHC staining and quantified (Figure 3A,B,C,D). The results showed that PQQ treatments significantly alleviated the reduction in the diameter of C2C12 myotubes and the increase of ROS levels induced by TNF-α (Figure 3E).
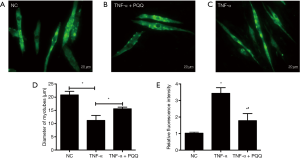
The expression level of MAFbx, MuRF-1 and MHC, which are dysregulated during muscle atrophy, were assessed by Western blotting. The expression level of MHC displayed remarkable decrease, and MAFbx and MuRF-1 displayed dramatically increase in C2C12 myotubes treated with TNF-α. PQQ treatments significantly prevented the decrease of MHC and the increase of MAFbx and MuRF-1 levels (Figure 4).
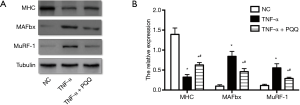
Discussion
Cachexia, a devastating, multifactorial and often irreversible syndrome, is most commonly observed in individuals with advanced cancer (1,2,29). Cachexia leads to substantial weight loss, primarily from loss of skeletal muscle and body fat. The characteristic sign of cachexia is muscle wasting, driven by inflammatory response, which significantly affects patient prognosis and increases mortality (5,30). To date, effective countermeasures have been still deficiency to alleviate muscle atrophy. It is well-known that inflammatory cytokines such as IL-1 beta, TNF-α, IL-6 and interferon-gamma have been involved in cancer cachectic response (31). Inflammatory mediators are key contributors to cancer cachexia, and they are the driver of skeletal muscle atrophy in cancer cachexia (6,32,33). Therefore, blocking inflammatory response was considered to be an effective treatment to prevent skeletal muscle atrophy under cachectic conditions. Cachexia is more common in malignant tumor, such as non-small cell cancer (34). TNF-α displayed an elevated expression level in sarcopenia and cancer cachexia and the elevated TNF-α is remarkably correlated with muscle wasting (35). Tsubouchi et al. suggested that inhibiting the inductions of TNF-α could ameliorate body weight loss in the mouse model of lung adenocarcinoma (36). Now, TNF-α is often used to induce cachexia associated C2C12 myotubes atrophy model (8-10).
Skeletal muscle atrophy is closely related to a reduced myofibrillar protein which results from both increased proteolysis and decreased protein synthesis, as evidenced by the reduced muscle fiber CSA and diameter (2). Normally, ROS content was at relatively low levels in skeletal muscle fibers under physiological conditions (23). Whereas, the increased ROS caused the increase in protein breakdown through upregulating the atrophy related factors such as MuRF1 and MAFbx (23,24). Although growing evidence has suggested that high levels of ROS might play a crucial role in muscle wasting, effective targeting therapies are still deficient for muscle atrophy.
Regarding this, the current study was the first to report that ROS generation was enhanced in atrophied skeletal muscle induced by cachexia, and increased ROS might play an important role in skeletal muscle atrophy induced by cachexia. Then, the function of increased ROS on the atrophic response was examined. NAC, free radical scavenger, was incubated with C2C12 myotubes treated with TNF-α. These results showed that NAC treatments significantly inhibited the reduction in the diameter of myotubes, as well as the increase in ROS production. Concomitantly, the decrease of the expression level of MHC and the increase of MAFbx and MuRF-1 levels were attenuated. These results were consistent with the previous reports that free radical scavenger NAC efficiently decreased the ROS levels and restored the expression level of MHC (27,37). Our data suggested that the increased ROS caused by cachexia played a central role in skeletal muscle atrophy.
PQQ is a water-soluble naturally occurring antioxidant component that has a strong anti-oxidant capacity. It not only serves as a mediator participating in redox reactions in the mitochondrial respiratory chain but also serves as a critical scavenger participating in scavenging ROS (38). Pretreated rats with PQQ could observably decrease ROS generation after ICH, which would be probably due to its antioxidant properties (39). PQQ could also significantly enhance the activities of superoxide dismutase, catalase and glutathione peroxidase (40). Therefore, in this study, we elucidated the potential of PQQ to ameliorate the muscle atrophy caused by cachexia. Our data demonstrated that the protective effect of PQQ on muscle atrophy was similar to that of NAC. PQQ treatments significantly alleviated the reduction in the diameter of myotubes and the increase of ROS content caused by cachexia, as well as preventing the decrease of MHC levels and the increase of MAFbx and MuRF-1 levels. Previous study indicated that PQQ could activate PGC-1α (41). PGC-1α expression could be useful to preserve muscle mass in functional denervation or aging (42). Overexpression of PGC-1α not only could block the TWEAK-induced atrophy program but also decrease the expression level of Fn14 in the skeletal muscle suffered from denervation (42). At the same time, TWEAK may activate the nuclear factor-κB (NF-κB) signaling pathway and the proteolytic pathways such as UPS and the ALP to induce muscle proteolysis (43). According to these, we might speculate that PQQ prevented the increase of MAFbx and MuRF-1 levels to relieve muscle atrophy by activating PGC-1α, and then blocking TWEAK. These data might be responsible for the explanation for PQQ preventing muscle atrophy. However, the exact molecular mechanism is still unclear.
Therefore, investigations into the signaling pathways that connect PQQ to cachexia induced muscle atrophy is still to be an important topic for future research, which might lead to the development of biological targets for therapeutic intervention to protect against skeletal muscle atrophy induced by cachexia.
Acknowledgements
Funding: This study was supported by National Natural Science Foundation of China (grant No. 81671230, 81301628), the 973 Program (grant No. 2014CB542202 and 2014CB542203), a project funded by Jiangsu Provincial Key Medical Center, the Priority Academic Program Development of Jiangsu Higher Education Institutions (PAPD), China Postdoctoral Science Foundation (grant No. 2016M591894), Jiangsu Postdoctoral Science Foundation (grant No. 1601040A), Fund of Doctoral Start-up of Nantong University (grant No. 15B18, 17ZZ040), Jiangsu College Students Innovation and Entrepreneurship Training Program (grant No. 201610304096X, 201710304029Z), Graduate Science and Technology Innovation Program (grant No. KYLX16-0965) and Nantong Science and Technology Innovation Program (grant No. MS12016008).
Footnote
Conflicts of Interest: The authors have no conflicts of interest to declare.
References
- Porporato PE, Filigheddu N, Reano S, et al. Acylated and unacylated ghrelin impair skeletal muscle atrophy in mice. J Clin Invest 2013;123:611-22. [PubMed]
- Fukawa T, Yan-Jiang BC, Min-Wen JC, et al. Excessive fatty acid oxidation induces muscle atrophy in cancer cachexia. Nat Med 2016;22:666-71. [Crossref] [PubMed]
- D’Costa NM, Chavez-Munoz C, So AI. Role of fibroblast growth factor receptor in sunitinib-resistant renal cell carcinoma. Biotarget 2017;1:3. [Crossref]
- Zhuang P, Zhang J, Wang Y, et al. Reversal of muscle atrophy by Zhimu and Huangbai herb pair via activation of IGF-1/Akt and autophagy signal in cancer cachexia. Support Care Cancer 2016;24:1189-98. [Crossref] [PubMed]
- Reed SA, Sandesara PB, Senf SM, et al. Inhibition of FoxO transcriptional activity prevents muscle fiber atrophy during cachexia and induces hypertrophy. FASEB J 2012;26:987-1000. [Crossref] [PubMed]
- Braun TP, Szumowski M, Levasseur PR, et al. Muscle atrophy in response to cytotoxic chemotherapy is dependent on intact glucocorticoid signaling in skeletal muscle. PLoS One 2014;9:e106489. [Crossref] [PubMed]
- Wu YY, Chao TY, Liu HY, et al. The correlation between a chronic inflammatory marker Tartrate-resistant acid phosphatase 5a with cancer cachexia. J BUON 2015;20:325-31. [PubMed]
- Hall DT, Ma JF, Marco SD, et al. Inducible nitric oxide synthase (iNOS) in muscle wasting syndrome, sarcopenia, and cachexia. Aging (Albany NY) 2011;3:702-15. [Crossref] [PubMed]
- Yuan L, Han J, Meng Q, et al. Muscle-specific E3 ubiquitin ligases are involved in muscle atrophy of cancer cachexia: an in vitro and in vivo study. Oncol Rep 2015;33:2261-8. [Crossref] [PubMed]
- Miao C, Lv Y, Zhang W, et al. Pyrrolidine Dithiocarbamate (PDTC) Attenuates Cancer Cachexia by Affecting Muscle Atrophy and Fat Lipolysis. Front Pharmacol 2017;8:915. [Crossref] [PubMed]
- Wan J, Chen D, Yu B, et al. Leucine Protects Against Skeletal Muscle Atrophy in Lipopolysaccharide-Challenged Rats. J Med Food 2017;20:93-101. [Crossref] [PubMed]
- Gatica D, Klionsky DJ. New insights into MTORC1 amino acid sensing and activation. Biotarget 2017;1:2. [Crossref]
- Zhao J, Zhai B, Gygi SP, et al. mTOR inhibition activates overall protein degradation by the ubiquitin proteasome system as well as by autophagy. Proc Natl Acad Sci U S A 2015;112:15790-7. [Crossref] [PubMed]
- Polge C, Attaix D, Taillandier D. Role of E2-Ub-conjugating enzymes during skeletal muscle atrophy. Front Physiol 2015;6:59. [Crossref] [PubMed]
- Wing SS. Deubiquitinating enzymes in skeletal muscle atrophy-An essential role for USP19. Int J Biochem Cell Biol 2016;79:462-8. [Crossref] [PubMed]
- Winbanks CE, Murphy KT, Bernardo BC, et al. Smad7 gene delivery prevents muscle wasting associated with cancer cachexia in mice. Sci Transl Med 2016;8:348ra98. [Crossref] [PubMed]
- He Q, Qiu J, Dai M, et al. MicroRNA-351 inhibits denervation-induced muscle atrophy by targeting TRAF6. Exp Ther Med 2016;12:4029-34. [Crossref] [PubMed]
- Sun H, Gong Y, Qiu J, et al. TRAF6 inhibition rescues dexamethasone-induced muscle atrophy. Int J Mol Sci 2014;15:11126-41. [Crossref] [PubMed]
- Sun H, Qiu J, Chen Y, et al. Proteomic and bioinformatic analysis of differentially expressed proteins in denervated skeletal muscle. Int J Mol Med 2014;33:1586-96. [Crossref] [PubMed]
- Qiu J, Fang Q, Xu T, et al. Mechanistic Role of Reactive Oxygen Species and Therapeutic Potential of Antioxidants in Denervation- or Fasting-Induced Skeletal Muscle Atrophy. Front Physiol 2018;9:215. [Crossref] [PubMed]
- Desgeorges MM, Devillard X, Toutain J, et al. Molecular mechanisms of skeletal muscle atrophy in a mouse model of cerebral ischemia. Stroke 2015;46:1673-80. [Crossref] [PubMed]
- Gomes AV, Waddell DS, Siu R, et al. Upregulation of proteasome activity in muscle RING finger 1-null mice following denervation. FASEB J 2012;26:2986-99. [Crossref] [PubMed]
- Fang Q, Xu T, Wu C, et al. Biotargets in Neural Regeneration. Biotarget 2017;1:6. [Crossref]
- Rodney GG, Pal R, Abo-Zahrah R. Redox regulation of autophagy in skeletal muscle. Free Radic Biol Med 2016;98:103-12. [Crossref] [PubMed]
- Kumar N, Kar A. Pyrroloquinoline quinone (PQQ) has potential to ameliorate streptozotocin-induced diabetes mellitus and oxidative stress in mice: A histopathological and biochemical study. Chem Biol Interact 2015;240:278-90. [Crossref] [PubMed]
- Sun H, Zhu T, Ding F, et al. Proteomic studies of rat tibialis anterior muscle during postnatal growth and development. Mol Cell Biochem 2009;332:161-71. [Crossref] [PubMed]
- Abrigo J, Rivera JC, Simon F, et al. Transforming growth factor type beta (TGF-beta) requires reactive oxygen species to induce skeletal muscle atrophy. Cell Signal 2016;28:366-76. [Crossref] [PubMed]
- Eruslanov E, Kusmartsev S. Identification of ROS using oxidized DCFDA and flow-cytometry. Methods Mol Biol 2010;594:57-72. [Crossref] [PubMed]
- Argiles JM, Busquets S, Stemmler B, et al. Cancer cachexia: understanding the molecular basis. Nat Rev Cancer 2014;14:754-62. [Crossref] [PubMed]
- Laviano A, Koverech A, Mari A. Cachexia: clinical features when inflammation drives malnutrition. Proc Nutr Soc 2015;74:348-54. [Crossref] [PubMed]
- Argiles JM, Busquets S, Lopez-Soriano FJ. Anti-inflammatory therapies in cancer cachexia. Eur J Pharmacol 2011;668 Suppl 1:S81-6. [Crossref] [PubMed]
- Navari RM. Overview of the neurokinin-1 receptor antagonists. Biotarget 2017;1:8. [Crossref]
- Li B, Wan L, Li Y, et al. Baicalin, a component of Scutellaria baicalensis, alleviates anorexia and inhibits skeletal muscle atrophy in experimental cancer cachexia. Tumour Biol 2014;35:12415-25. [Crossref] [PubMed]
- LeBlanc TW, Nipp RD, Rushing CN, et al. Correlation between the international consensus definition of the Cancer Anorexia-Cachexia Syndrome (CACS) and patient-centered outcomes in advanced non-small cell lung cancer. J Pain Symptom Manage 2015;49:680-9. [Crossref] [PubMed]
- Sharples AP, Polydorou I, Hughes DC, et al. Skeletal muscle cells possess a 'memory' of acute early life TNF-alpha exposure: role of epigenetic adaptation. Biogerontology 2016;17:603-17. [Crossref] [PubMed]
- Tsubouchi H, Yanagi S, Miura A, et al. Ghrelin relieves cancer cachexia associated with the development of lung adenocarcinoma in mice. Eur J Pharmacol 2014;743:1-10. [Crossref] [PubMed]
- Wang X, Li H, Zheng A, et al. Mitochondrial dysfunction-associated OPA1 cleavage contributes to muscle degeneration: preventative effect of hydroxytyrosol acetate. Cell Death Dis 2014;5:e1521. [Crossref] [PubMed]
- Nakano M, Kawasaki Y, Suzuki N, et al. Effects of Pyrroloquinoline Quinone Disodium Salt Intake on the Serum Cholesterol Levels of Healthy Japanese Adults. J Nutr Sci Vitaminol (Tokyo) 2015;61:233-40. [Crossref] [PubMed]
- Lu H, Shen J, Song X, et al. Protective Effect of Pyrroloquinoline Quinone (PQQ) in Rat Model of Intracerebral Hemorrhage. Cell Mol Neurobiol 2015;35:921-30. [Crossref] [PubMed]
- Guan S, Xu J, Guo Y, et al. Pyrroloquinoline quinone against glutamate-induced neurotoxicity in cultured neural stem and progenitor cells. Int J Dev Neurosci 2015;42:37-45. [Crossref] [PubMed]
- Kuo YT, Shih PH, Kao SH, et al. Pyrroloquinoline Quinone Resists Denervation-Induced Skeletal Muscle Atrophy by Activating PGC-1alpha and Integrating Mitochondrial Electron Transport Chain Complexes. PLoS One 2015;10:e0143600. [Crossref] [PubMed]
- Hindi SM, Mishra V, Bhatnagar S, et al. Regulatory circuitry of TWEAK-Fn14 system and PGC-1alpha in skeletal muscle atrophy program. FASEB J 2014;28:1398-411. [Crossref] [PubMed]
- Sato S, Ogura Y, Kumar A. TWEAK/Fn14 Signaling Axis Mediates Skeletal Muscle Atrophy and Metabolic Dysfunction. Front Immunol 2014;5:18. [Crossref] [PubMed]