Expression levels of paraoxonase-1 in aortic valve tissue are associated with the progression of calcific aortic valve stenosis
Introduction
Calcific aortic valve stenosis (CAVS) represents a late stage in the progression of calcific aortic valve disease (CAVD) (1). Associated symptoms develop rapidly with age, and patients with severe CAVS have an extremely high risk of sudden death. Therefore, CAVS has become one of the main health problems in both the Chinese and Western populations in recent years (2). To date, however, no drugs or treatments can effectively prevent or change the development of this disease (3).
CAVS was previously considered to be a passive and degenerative process of aging. However, recent research has confirmed that aortic valve calcification is an active and highly regulated automatic pathophysiologic process involving a mechanism similar to that of coronary atherosclerosis (4,5), a chronic process of inflammatory reactivity and disordered lipid metabolism (6). Calcified aortic stenosis and atherosclerosis share many common pathologic features, including a close relationship between the level of circulating oxidized low-density lipoprotein (ox-LDL) and the extent of calcification-mediated valve remodeling (7). When valve endothelial cells are subjected to mechanical stress, monocyte-derived macrophages present in the subintimal space and valve endothelium avidly take up ox-LDL to become foam cells, which play a key role in the development of CAVS (8,9).
Paraoxonase-1 (PON1) belongs to the paraoxonase multigene family, whose genes are located adjacent to each other on chromosome 7q21-22 in humans (10). Importantly, PON1 inhibits the oxidation of LDL and cell membrane lipids (9,11), and therefore has anti-atherosclerotic and anti-inflammatory properties. Mackness et al. and Watson et al. suggested that PON1 could retard the development of atherosclerosis (12,13). Epidemiologically, the serum levels of PON1 were found to be inversely proportional to the levels of oxidized fatty acids (14). Mice with a genetic knockout of PON1 are more prone to oxidant stress than normal mice (15).
Our previous results suggested that a functional single nucleotide polymorphism (SNP) in the 3' untranslated region of PON1 is associated with the risk of CAVS in humans (16). However, none of the abovementioned studies quantitatively detected PON1 expression in human aortic valve tissue. This is the first study to detect PON1 expression specifically in human aortic valve tissue, and to illustrate a significant relationship between PON1 level and aortic valve calcification in detail.
Methods
Study population
We screened 118 consecutive patients with CAVS who underwent aortic valve replacement (with or without aortic surgery) at our center from October 2016 to December 2017. The patients’ conditions met the diagnostic criteria of the American College of Cardiology and American Heart Association. Thirty-five patients with Marfan syndrome, aortic dissection (Stanford A), or simple aortic insufficiency, who were treated at our department during the same period, were included as a control group. We divided the patients according to the maximum aortic valve pressure gradient (PGmax), determined from echocardiography results, into the mild stenosis (n=39, PGmax <50 mmHg), moderate stenosis (n=40, PGmax 50 to <70 mmHg), and severe stenosis (n=39, PGmax ≥70 mmHg) groups. Patients with a history of rheumatic disease, mitral/tricuspid valve diseases, valve stenosis and/or moderate-to-severe aortic insufficiency or left ventricular ejection fraction (LVEF) <40%, endocarditis, atrial fibrillation, malignant systemic tumor, hematologic disease (including leukemia), acute or chronic infection, or inflammatory conditions were excluded from the study. The protocol was approved by the Institutional Committee on Clinical Investigation of Shandong Provincial Hospital affiliated to Shandong University, and informed consent was obtained from all patients.
Laboratory blood evaluation
Peripheral blood samples for testing were collected in the morning from fasted patients. The blood biochemical index, including LDL and high-density lipoprotein (HDL), were evaluated using an ADVIA-2400 biochemical analyzer (Siemens, Munich, Germany) with matching reagents.
Immunohistochemical analysis
Fresh aortic valve tissue samples were collected from the hearts of case and control patients and stored in 4% formaldehyde for 30 min. Subsequently, the specimens were fixed in paraffin, routinely sliced, and subjected to decalcification of the fixed valves before immunohistochemical analysis. PON1 protein expression was detected qualitatively in the aortic valve tissue through immunohistochemical analysis. A PON1-specific polyclonal antibody was obtained by inoculating rabbits with peptides derived from specific sequences of mature PON1 and used at a dilution of 1:100 (Invitrogen/Thermo Fisher Scientific Company, Carlsbad, CA, USA; cat. no. PA5-28997). The following procedure was applied. Prepared pathologic sections of aortic valve tissue were: (I) baked in an oven at 60 °C for 1.5 h, (II) followed by dewaxing continuously through a series of xylene, anhydrous ethanol, and 95% ethanol. Next, the sections were (III) washed in distilled water and then phosphate-buffered saline (PBS), and (IV) subjected to antigen repair in a sodium citrate solution that was heated in a pressure cooker (2,100 W, followed by a downshift to 800 W) for 21 min. (V) After washing the sections in PBS, the endogenous peroxidases were blocked. (VI) The sections were incubated for 18 h in a PON1 polyclonal antibody at 4 °C in a dark, humid environment. (VII) After washing in PBS, the sections were treated with horseradish peroxidase-conjugated goat anti-rabbit IgG for 30 min at 25 °C in a dark, humid environment, followed by (VIII) incubation with 3,3'-diaminobenzidine tetrahydrochloride chromogen for 15 s. (IX) Finally, the pathologic sections were stained with hematoxylin, processed with 1% hydrochloric acid alcohol, dried, and observed under an optical microscope.
Enzyme-linked immunosorbent assay (ELISA)
The same samples were initially stored in liquid nitrogen and transferred to a −80 °C freezer after 30 min. The decalcification, tissue cleaning, and preliminary treatment steps are important in this assay.
We first repeatedly washed the valve tissue with sterile PBS. Next, we cut the same leaflet tissue weight (~0.5 g) and generated a tissue cell suspension through grinding and ultrasonic cell disruption. We then centrifuged the specimen for 10 min at 1,000 ×g to obtain a supernatant. An ELISA kit specific for human PON1 [Shanghai FuSheng Industrial Co., Ltd., Shanghai, China; intra-assay coefficient of variation (CV): 7.6%, inter-assay CV: 9.4%] was used to detect the level of PON1 in all samples, according to the manufacturer’s instructions (standard dilution, sampling, incubation, combination, washing, enzymatic treatment, incubation, washing, development, termination reaction; detection and calculation were performed using a THERMO Multiskan FC microplate (Thermo Fisher Scientific Company) reader at a wavelength of 450 nm). Figure 1 depicts the standard curve for PON1 levels (R2 >0.99, Y-intercept =0.017).
Echocardiographic measurement
Comprehensive transthoracic echocardiograms were obtained using an echocardiography instrument [Philips IE33 with an s5-1 (2.5–3.5 Hz) transducer; Philips Electronics Ltd., Eindhoven, The Netherlands] at the Department of Ultrasound of our institution. All evaluators, including the echocardiogram technologists and interpreters, were blinded to the patients’ treatment status. Standard Doppler parameters, including ejection fraction (determined using the modified Simpson method), left ventricular end-diastolic diameter, aortic maximal jet velocity, and PGmax, were recorded.
Statistical analysis
Data were collected and analyzed using SPSS (version 19.0; IBM Corp., Armonk, NY, USA). Continuous variables are presented as means ± standard deviations, and categorical variables are expressed as frequencies and percentages. One-way analysis of variance was used to compare the continuous numeric parameters of each group, and the chi-square test was used to compare the categorical variables. Pearson correlation analysis was performed to examine the relationship between the PON1 level and PGmax.
Results
The baseline characteristics and laboratory parameters of the study groups are presented in Table 1. The case and control groups were similar with respect to age (56.63±7.94 vs. 53.29±12.38 years; P=0.060), sex distribution (male: 70.3% vs. 62.9%; P=0.740), alcohol consumption frequency (49.2% vs. 34.3%; P=0.110), prevalence of hypertension (66.1% vs. 77.1%; P=0.090), and prevalence of diabetes mellitus (52.5% vs. 37.1%; P=0.235). The case group had a significantly higher prevalence of smoking than the control group (62.7% vs. 42.9%; P=0.037). Moreover, the case group had a significantly lower HDL level (1.12±0.30 vs. 1.42±0.33 mmol/L; P<0.001) and a significantly higher LDL level (2.64±0.69 vs. 2.21±0.58 mmol/L; P<0.001) than the control group. The echocardiographic assessment revealed a significantly higher maximum forward velocity (324.57±126.53 vs. 155.40±41.92 cm/s; P<0.001) and maximum gradient (56.70±33.39 vs. 14.49±5.53 mmHg; P<0.001) in the case group than in the control group. The LVEF of the case group was significantly lower than that of the control group (56.28%±7.03% vs. 59.80%±3.51%; P=0.005). In tissue samples, positive immunohistochemical staining for PON1 yielded a dark brown color (Figure 2). Typical pathologic sections from the case and control groups are shown in Figure 3. Simultaneously, we observed a significantly lower level of PON1 in tissues from the case group than in those from the control group (124.31±19.67 vs. 243.81±44.21 nmol/L; P<0.001) (Figure 4).
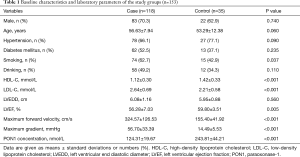
Full table
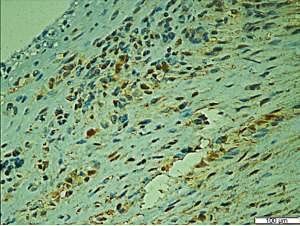
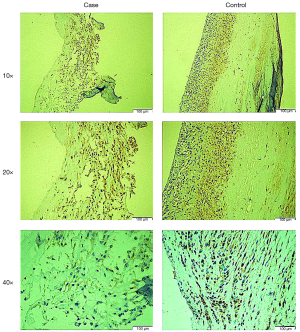
Table 2 presents the results of subgroup analysis. Statistically significant differences in all parameters were observed among the three groups (P value <0.05). There were no statistically significant differences between the mild and moderate stenosis groups except in LVEF (58.90%±3.80% vs. 55.88%±6.35%; P=0.012), maximum forward velocity (214.18±59.77 vs. 270.93±68.72 cm/s; P<0.001), and maximum gradient (24.79±11.25 vs. 47.53±13.29 mmHg; P<0.001). Remarkably, however, the PON1 level in the mild and moderate groups was significantly higher than that in the severe group [130.66±15.13 (mild) and 128.14±10.83 nmol/L (moderate) vs. 105.28±15.98 nmol/L (severe); P<0.001 for both], as illustrated in Figure 5. Pearson correlation analysis indicated that the maximum gradient exhibited a moderate and negative correlation with the PON1 level in the case group (r=0.43, P<0.001) (Figure 6).
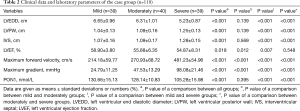
Full table
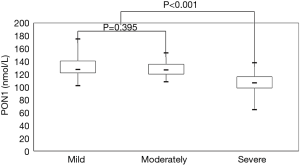
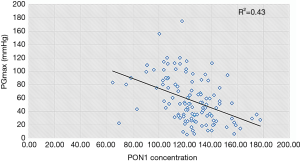
Discussion
The increasing depth of research on CAVD has clarified that the development of CAVS is attributable to a series of interactive steps, such as inflammation, endothelial-to-mesenchymal transition, matrix vesicle formation and microcalcification, extracellular matrix remodeling, angiogenesis and neo-vascularization, and lipid metabolism disorder (7,17). In the context of either a normal cholesterol level or hypercholesterolemia and atherosclerosis, endothelial dysfunction leads to lipid deposition in aortic valve interstitial cells (VICs). Studies have shown that elevated levels of circulating proatherogenic ox-LDL can be detected in patients with significant fibrocalcific aortic valve disease (18). Lower levels of atheroprotective HDL are observed in calcified human aortic valves, which is a dysfunctional characteristic, as demonstrated by a decrease in the activity of the HDL-associated antioxidant enzyme PON1 (19,20). Cagirci et al. detected lower serum PON1 activity in patients with CAVS by using spectrophotometry and found that this phenomenon positively correlated with disease severity. Similarly, in our study, we observed significantly greater areas and densities of immunofluorescence staining in tissue samples from patients with aortic stenosis than in patients from the control group (Figure 3). An analysis at a higher magnification revealed that those stained cells were almost all VICs (Figure 2), consistent with our hypothesis and the results of previous studies. To our knowledge, this is the first study to evaluate the association between the PON1 level in valve tissue and the severity of CAVS.
In mammals, the PON family is encoded by three distinct genes. Human PON genes are located on the long arm of the 7th chromosome (7q21.3–q22.1). PON1 and PON3 are synthesized in the liver and can be detected in the plasma, whereas PON2 is primarily an intracellular enzyme (21). PON1, which contains two calcium ions, is a 354-amino-acid-long calcium-dependent enzyme with a molecular mass of 43 kDa (22). Although the endogenous substrate of PON1 is not known, researchers speculate that its main substrates are lactones. In other words, PON1 catalyzes the reverse lactonization reaction, and its arylesterase and organophosphatase activities seem to be secondary (23,24). In recent years, many studies have confirmed that overexpression of PON1 can reduce the incidence of coronary atherosclerotic heart disease (25,26) via the following possible mechanisms: (I) inhibiting the oxidation of reactive oxygen to LDL and then reducing the content of ox-LDL, (II) increasing the cholesterol efflux from macrophages, and (III) acting on endothelial cells to reduce the synthesis of monocyte chemoattractant protein-1. In addition, in 2013, Aviram and Vaya demonstrated that PON1 knockout mice exhibited an extreme increase in the oxidative effects of macrophages and a decrease in the level of glutathione. Furthermore, mice deficient in PON1 and ApoE protein were found to be more likely to develop coronary atherosclerosis (27).
The exceptional similarity between CAVS and atherosclerosis laid the groundwork for our study. According to data, mechanical stress-induced endothelial cell damage allows a large number of macrophages to break through the endothelial cell layer and enter the valve interstitium to form foam cells via vascular cell adhesion molecule-1 and intracellular cell adhesion molecule-1. These foam cells stimulate the cytokines tissue necrosis factor-α, interleukin-1/-6, and transforming growth factor-β to activate the quiescent VICs via the transduction of Notch-1 and activation of α-smooth muscle actin, leading to the further development of bone-forming cells via various cellular regulatory pathways and ultimately to calcification of valve tissue (5,17,28,29). In the above-described process, large amounts of lipids can be detected in the blood circulation and valve tissues (7). Several studies have shown that PON1 can bind to HDL to inhibit the peroxide oxidation of LDL while stimulating the HDL-mediated efflux of cholesterol from macrophages (30). Therefore, an SNP in PON1 is associated with the occurrence of CAVS. A local reduction in PON1 levels in valve tissues leads to the loss of the anti-LDL peroxidation effect and prompts the conversion of VICs.
Most previous studies reported an association of the serum PON1 level with most atherosclerosis-like diseases (31-33). However, Marsillach et al. reported the downregulation of PON1 protein expression in smooth muscle cells during the development of atherosclerosis (34). A study on the involvement of PON1 in lipid metabolism or in a local cell signaling transduction pathway in valve tissues primarily relies on the ability to verify PON1 protein expression in valve tissues. Our study is the first of its kind to particularly evaluate aortic valve tissues, and the experiments confirmed our conjecture. Specifically, we observed downregulated PON1 protein expression in valve tissues from patients with CAVS, and the level of this protein correlated moderately and negatively with the PGmax. Accordingly, the PON1 level provides a certain degree of reference for evaluations of the PGmax, sudden death rate, and surgical risk in patients with CAVS. However, these results require further confirmation in a larger sample and with other experimental techniques.
Although PON1 was detected in valve tissues by using immunohistochemistry and ELISA, in situ hybridization was not used because of the difficulty in obtaining and preserving the tissue specimens. Therefore, it remains unclear whether PON1 is involved in the local metabolism of VICs and, particularly, to which cell signaling transduction pathways it is involved. Our next study will address these issues.
The levels of PON1 in serum and aortic valve tissue were treated as an entitative protective factor that is susceptible to many other factors. The local or circulating PON1 level could be potentially increased in high-risk patients to reduce the incidence or block the progression of CAVS.
In conclusion, we have pioneered the detection of PON1 in aortic valve tissues and illustrated in detail the relationship between the PON1 level and the severity of CAVS. PON1 is a protective protein, and the PON1 level in valve tissue appears to decrease as the degree of stenosis increases.
Acknowledgments
Funding: This work was supported by the Science and Technology Department of Shandong Province (No. 2016GSF201039, ZR2017BH017, and ZR2018BH002).
Footnote
Conflicts of Interest: The authors have no conflicts of interest to declare.
Ethical Statement: The authors are accountable for all aspects of the work in ensuring that questions related to the accuracy or integrity of any part of the work are appropriately investigated and resolved. The protocol was approved by the Institutional Committee on Clinical Investigation of Shandong Provincial Hospital affiliated to Shandong University (No. 2017548), and informed consent was obtained from all patients.
References
- Yutzey KE, Demer LL, Body SC, et al. Calcific aortic valve disease: a consensus summary from the Alliance of Investigators on Calcific Aortic Valve Disease. Arterioscler Thromb Vasc Biol 2014;34:2387-93. [Crossref] [PubMed]
- Lindroos M, Kupari M, Heikkila J, et al. Prevalence of aortic valve abnormalities in the elderly: an echocardiographic study of a random population sample. J Am Coll Cardiol 1993;21:1220-5. [Crossref] [PubMed]
- Thanassoulis G, Campbell CY, Owens DS, et al. Genetic associations with valvular calcification and aortic stenosis. N Engl J Med 2013;368:503-12. [Crossref] [PubMed]
- Chen JH, Simmons CA. Cell-matrix interactions in the pathobiology of calcific aortic valve disease: critical roles for matricellular, matricrine, and matrix mechanics cues. Circ Res 2011;108:1510-24. [Crossref] [PubMed]
- Dweck MR, Boon NA, Newby DE. Calcific aortic stenosis: a disease of the valve and the myocardium. J Am Coll Cardiol 2012;60:1854-63. [Crossref] [PubMed]
- Stewart BF, Siscovick D, Lind BK, et al. Clinical factors associated with calcific aortic valve disease. Cardiovascular Health Study. J Am Coll Cardiol 1997;29:630-4. [Crossref] [PubMed]
- Ruiz JL, Hutcheson JD, Aikawa E. Cardiovascular calcification: current controversies and novel concepts. Cardiovasc Pathol 2015;24:207-12. [Crossref] [PubMed]
- Camps J, Marsillach J, Joven J. The paraoxonases: role in human diseases and methodological difficulties in measurement. Crit Rev Clin Lab Sci 2009;46:83-106. [Crossref] [PubMed]
- Reddy ST, Devarajan A, Bourquard N, et al. Is it just paraoxonase 1 or are other members of the paraoxonase gene family implicated in atherosclerosis? Curr Opin Lipidol 2008;19:405-8. [Crossref] [PubMed]
- Aviram M, Rosenblat M. Paraoxonases 1, 2, and 3, oxidative stress, and macrophage foam cell formation during atherosclerosis development. Free Radic Biol Med 2004;37:1304-16. [Crossref] [PubMed]
- Mastorikou M, Mackness B, Liu Y, et al. Glycation of paraoxonase-1 inhibits its activity and impairs the ability of high-density lipoprotein to metabolize membrane lipid hydroperoxides. Diabet Med 2008;25:1049-55. [Crossref] [PubMed]
- Mackness B, Quarck R, Verreth W, et al. Human paraoxonase-1 overexpression inhibits atherosclerosis in a mouse model of metabolic syndrome. Arterioscler Thromb Vasc Biol 2006;26:1545-50. [Crossref] [PubMed]
- Watson AD, Berliner JA, Hama SY, et al. Protective effect of high density lipoprotein associated paraoxonase. Inhibition of the biological activity of minimally oxidized low density lipoprotein. J Clin Invest 1995;96:2882-91. [Crossref] [PubMed]
- Bhattacharyya T, Nicholls SJ, Topol EJ, et al. Relationship of paraoxonase 1 (PON1) gene polymorphisms and functional activity with systemic oxidative stress and cardiovascular risk. JAMA 2008;299:1265-76. [Crossref] [PubMed]
- Shih DM, Gu L, Xia YR, et al. Mice lacking serum paraoxonase are susceptible to organophosphate toxicity and atherosclerosis. Nature 1998;394:284-7. [Crossref] [PubMed]
- Wang Z, Chen S, Zhu M, et al. Functional SNP in the 3'UTR of PON1 is Associated with the Risk of Calcific Aortic Valve Stenosis via MiR-616. Cell Physiol Biochem 2018;45:1390-8. [Crossref] [PubMed]
- Leopold JA. Cellular mechanisms of aortic valve calcification. Circ Cardiovasc Interv 2012;5:605-14. [Crossref] [PubMed]
- Côté C, Pibarot P, Despres JP, et al. Association between circulating oxidised low-density lipoprotein and fibrocalcific remodelling of the aortic valve in aortic stenosis. Heart 2008;94:1175-80. [Crossref] [PubMed]
- Cagirci G, Cay S, Karakurt O, et al. Paraoxonase activity might be predictive of the severity of aortic valve stenosis. J Heart Valve Dis 2010;19:453-8. [PubMed]
- Lommi JI, Kovanen PT, Jauhiainen M, et al. High-density lipoproteins (HDL) are present in stenotic aortic valves and may interfere with the mechanisms of valvular calcification. Atherosclerosis 2011;219:538-44. [Crossref] [PubMed]
- Kulka M. A review of paraoxonase 1 properties and diagnostic applications. Pol J Vet Sci 2016;19:225-32. [Crossref] [PubMed]
- Précourt LP, Amre D, Denis MC, et al. The three-gene paraoxonase family: physiologic roles, actions and regulation. Atherosclerosis 2011;214:20-36. [Crossref] [PubMed]
- Jakubowski H, Zhang L, Bardeguez A, et al. Homocysteine thiolactone and protein homocysteinylation in human endothelial cells: implications for atherosclerosis. Circ Res 2000;87:45-51. [Crossref] [PubMed]
- Macharia M, Hassan MS, Blackhurst D, et al. The growing importance of PON1 in cardiovascular health: a review. J Cardiovasc Med (Hagerstown) 2012;13:443-53. [Crossref] [PubMed]
- Hernández-Díaz Y, Tovilla-Zárate CA, Juárez-Rojop IE, et al. Effects of paraoxonase 1 gene polymorphisms on heart diseases: Systematic review and meta-analysis of 64 case-control studies. Medicine (Baltimore) 2016;95:e5298. [Crossref] [PubMed]
- Chistiakov DA, Melnichenko AA, Orekhov AN, et al. Paraoxonase and atherosclerosis-related cardiovascular diseases. Biochimie 2017;132:19-27. [Crossref] [PubMed]
- Aviram M, Vaya J. Paraoxonase 1 activities, regulation, and interactions with atherosclerotic lesion. Curr Opin Lipidol 2013;24:339-44. [Crossref] [PubMed]
- Mathieu P, Boulanger MC. Basic mechanisms of calcific aortic valve disease. Can J Cardiol 2014;30:982-93. [Crossref] [PubMed]
- Pawade TA, Newby DE, Dweck MR. Calcification in Aortic Stenosis: The Skeleton Key. J Am Coll Cardiol 2015;66:561-77. [Crossref] [PubMed]
- Gugliucci A, Menini T. Paraoxonase 1 and HDL maturation. Clin Chim Acta 2015;439:5-13. [Crossref] [PubMed]
- Liu T, Zhang X, Zhang J, et al. Association between PON1 rs662 polymorphism and coronary artery disease. Eur J Clin Nutr 2014;68:1029-35. [Crossref] [PubMed]
- de Jesus Inês E, Sampaio Silva ML, de Souza JN, et al. Alterations in serum paraoxonase-1 activity and lipid profile in chronic alcoholic patients infected with Strongyloides stercoralis. Acta Trop 2017;166:1-6. [Crossref] [PubMed]
- Chernyavskiy I, Veeranki S, Sen U, et al. Atherogenesis: hyperhomocysteinemia interactions with LDL, macrophage function, paraoxonase 1, and exercise. Ann N Y Acad Sci 2016;1363:138-54. [Crossref] [PubMed]
- Marsillach J, Camps J, Beltran-Debón R, et al. Immunohistochemical analysis of paraoxonases-1 and 3 in human atheromatous plaques. Eur J Clin Invest 2011;41:308-14. [Crossref] [PubMed]