Insulin resistance, glucose intolerance and diabetes mellitus in obstructive sleep apnoea
Obstructive sleep apnoea (OSA) is a clinical syndrome characterized by repeated episodes of pharyngeal obstruction during sleep that lead to intermittent hypoxia (IH), sleep fragmentation and excessive daytime sleepiness. It is a highly prevalent disorder affecting about 14% of men and 5% of women and its prevalence is rapidly rising due to the strong association of OSA with obesity (1). The major health burden in OSA patients is an increased risk of cardiovascular diseases, such as systemic arterial hypertension, coronary artery disease, heart failure and stroke, an association which is corroborated by numerous large-scale epidemiological and prospective studies (2). Furthermore, there is increasing evidence of an independent association of OSA with metabolic dysfunction, and in particular with alterations in glucose metabolism. Subjects with OSA seem to be at greater risk of developing type 2 diabetes mellitus (T2DM), insulin resistance (IR) and metabolic syndrome (MetS), an association which seems to be at least in part irrespective of the degree of obesity. Indeed, OSA and obesity may exert synergistic negative effects on glucose metabolisms. However, studies in this field have yielded inconsistent results, and the impact of OSA treatment on glucose metabolism and metabolic dysfunction is also not clearly established.
In this article, we will review the current evidence evaluating potential links between OSA and T2DM, IR and MetS, including the potential impact of continuous positive airway pressure (CPAP) treatment on these consequences. We will further discuss the possible mechanisms underlying metabolic dysfunction in OSA, which include several pathophysiological pathways triggered by the characteristic features of OSA, namely sleep disturbance and IH.
Clinical and epidemiological data
Association between obstructive sleep apnoea (OSA) and type 2 diabetes mellitus (T2DM)
OSA in diabetic cohorts
Given their shared relationship with obesity, a substantial burden of sleep disordered breathing in diabetic cohorts and a high prevalence of T2DM in OSA populations is perhaps to be expected. However, there is increasing evidence that the two disorders may have an independent link, one beyond a simple shared association with obesity. OSA is certainly highly prevalent in patients with T2DM. When 240 subjects attending British hospital- and community-based diabetes clinics underwent domiciliary overnight oximetry, with confirmatory cardio-respiratory sleep studies performed on those whose oximetry was suggestive of sleep disordered breathing, 23% were found to have OSA [defined as an oxygen desaturation index (ODI) >10/h] (3). An even greater prevalence of OSA was found among 305 obese American diabetics evaluated using home polysomnography (PSG). In this cohort, only 13.4% did not have sleep disordered breathing, with 22.6% classified as severe OSA (4). A key explanatory factor for the discrepancy between these two studies was the degree of obesity within their cohorts—subjects in the US-based Sleep-AHEAD study had a mean body mass index (BMI) of 36.1 kg/m2, compared with 28.8 kg/m2 in the English group.
Prevalence of T2DM in OSA cohorts
Diabetes is also over-represented in patients with OSA when compared with the general population (Table 1). The most useful data in this regard is derived from large community-based studies. In the Wisconsin sleep cohort study, physician-diagnosed T2DM was present in 1.5% of participants with an apnoea/hypopnea index (AHI) <5, but 7.8% with an AHI ≥15 (5). Similarly, 8.8% of men and 7.7% of women with an AHI <5 in the Sleep Heart Health Study had known T2DM, compared with 16.9% and 16.7% respectively of those with an AHI ≥30 (14). In a large Canadian sleep clinic cohort, 4.4% without OSA had an antecedent diagnosis of diabetes, vs. 15.3% with severe OSA (6).
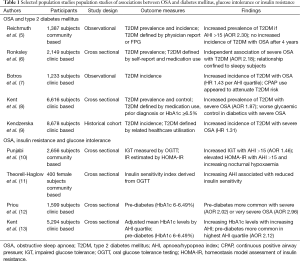
Full table
The increased prevalence of T2DM in patients with more severe OSA is not completely explained by traditional risk factors for metabolic disease. In a community-based analysis of 1,387 subjects of the Wisconsin Sleep Cohort, 23% of whom had an AHI ≥5, moderate-severe OSA was independently associated with prevalent physician-diagnosed T2DM, despite adjustment for age, gender, and body habitus [adjusted odds ratio (OR) =2.30; 95% CI, 1.28-4.11] (5). This relationship was still stronger in those with an AHI ≥30 (adjusted OR =3.48; 95% CI, 1.69-7.18). Among 2,149 patients referred to Canadian sleep clinics for diagnostic testing, severe OSA conferred an adjusted OR of 1.82 (95% CI, 1.07-3.10) for concomitant T2DM (6). In this study diabetes was defined according to self-report, physician diagnosis, medication usage, or from administrative database records. Perhaps surprisingly, this relationship appeared to be confined to sleepy subjects—those with an Epworth Sleepiness Score (ESS) score ≥10 had an adjusted OR of 2.59 (95% CI, 1.35-4.97) for prevalent diabetes, as compared with 1.16 (95% CI, 0.31-4.37) for those without subjective daytime sleepiness.
An important limitation of population-level studies of T2DM prevalence in sleep disordered breathing is their reliance on patient self-reporting, administrative databases or fasting plasma glucose measurement to establish a diagnosis of T2DM. The European Sleep Apnoea Cohort (ESADA) study is a multi-centre, multinational study involving over 15,000 patients attending sleep laboratories across Europe, Israel and Turkey. Participants in ESADA are screened at enrolment with glycosylated haemoglobin (HbA1c) measurement, which has recently been approved as a stand-alone diagnostic test for T2DM (15), and may identify a higher proportion of diabetics in sleep cohorts than reliance on fasting plasma glucose (16). In an analysis of nearly 7,000 subjects in the ESADA cohort, a diagnosis of severe sleep disordered breathing was associated with a markedly increased likelihood of prevalent T2DM, following adjustment for obesity, age, co-morbidities and medication use (adjusted OR =1.87; 95% CI, 1.45-2.42) (8).
Similarly, data from smaller, clinic-based studies using more robust methods to detect occult hyperglycaemia support an independent relationship between OSA and T2DM. In a French study of 595 men with suspected OSA undergoing PSG and subsequent oral glucose tolerance testing (OGTT), T2DM was found in 30.1% of subjects with an AHI >10, compared with 13.9% of non-apnoeic snorers (17). In a multivariate linear regression model, AHI was an independent negative predictor of insulin sensitivity. In a similar study, 129 Japanese patients with newly diagnosed OSA underwent OGTT; a post-load glucose in the diabetic range was found in 33.8% with an AHI ≥30 vs. 15% with an AHI <15 (18). In both of these studies, a large proportion of subjects had undiagnosed T2DM, emphasising the importance of rigorous screening in clinical and epidemiological studies.
Incidence of T2DM in OSA cohorts
There is some discordance in the published literature on how OSA may predict the subsequent development of T2DM. Studies evaluating snorers, performed largely without objective assessment of sleep-disordered breathing, are suggestive of a link. A questionnaire-based study of 2,504 Swedish men aged 30-69 assessed the relationship of self-reported snoring with development of self-reported T2DM over a 10-year period (19). The 2.4% of non-snorers developed T2DM over the study period, compared with 5.4% of snorers. In an analysis confined to obese participants, 13.5% of snorers and 8.6% of non-snorers reported incident T2DM. A complimentary study of 69,852 US female nurses found regular snorers had an adjusted OR of 2.02 (95% CI, 1.71-2.40) for incident T2DM over a decade of follow-up compared with non-snorers, following adjustment for obesity, age and other risk factors for metabolic disease (20).
Few studies have longitudinally evaluated if objectively measured sleep disordered breathing constitutes an independent risk factor for T2DM. The 26 new cases of T2DM occurred among 978 participants in the Wisconsin sleep cohort over 4 years of follow-up, and in unadjusted analysis moderate-severe OSA was associated with an increased likelihood of incident diabetes mellitus (OR =4.06; 95% CI, 1.86-8.85) (5). This relationship was abolished following adjustment for age, gender, and body habitus (adjusted OR =1.62; 95% CI, 0.67-3.65; P=0.24), and no alteration in risk of T2DM was seen in those commenced on CPAP treatment. Conversely, in a study of 544 subjects referred for assessment of suspected sleep disordered breathing with a mean duration of follow-up of 2.7 years, T2DM occurred at a rate of 5.5 cases per 100 patient years in OSA patients, as opposed to 1.8 cases per 100 patient years in non-apnoeic snorers (7). This relationship survived adjustment for confounding variables [adjusted hazard ratio (HR) per AHI quartile =1.43; 95% CI, 1.10-1.86), and CPAP significantly attenuated the risk of incident T2DM (HR =0.53; 95% CI, 0.28-0.99).
Similarly, a recent historical cohort study, evaluating over 8,000 non-diabetic Canadian subjects attending a sleep laboratory, found that after a median follow-up period of 67 months, and following statistical adjustment for confounding factors, subjects with severe OSA were 30% more likely to develop clinically overt T2DM than those with an AHI <5 (9).
Glycaemic control in subjects with diabetes mellitus and obstructive sleep apnoea (OSA)
The presence of sleep disordered breathing may also contribute to poor diabetic control. In a study of 60 US patients recruited from a hospital diabetes clinic, 77% were found to have OSA (21). Increasing OSA severity was associated with increased HbA1c levels after adjustment for demographic, anthropometric and clinical variables, with an adjusted mean HbA1c level 3.69% higher among diabetics in the severe OSA group than in the non-apnoeic group. HbA1c also correlated significantly in multivariate linear regression analysis with AHI and other indices of sleep disordered breathing.
Among over 1,100 subjects with T2DM participating in the ESADA study, increasing OSA severity predicted increased likelihood of suboptimal glycaemic control (HbA1c >7%) (22). When compared with non-apnoeic diabetic patients, those with severe OSA had an adjusted OR of 2.02 (95% CI, 1.11-3.66) for poor diabetic control, following adjustment for confounding factors including prescription of diabetic medications (8). Similarly, adjusted mean HbA1c levels among diabetic patients increased with increasing OSA severity, from 6.76% (95% CI, 6.39-7.13) in those with no OSA to 7.48% (95% CI, 7.18-7.79) in those with severe OSA.
Treatment of obstructive sleep apnoea (OSA) and glycaemic control
The ability of CPAP therapy to make a meaningful impact upon diabetic control remains unclear (Table 2). Insulin sensitivity, as measured by hyperinsulinaemic euglycaemic clamp, improved significantly from 11.4±6.2 to 15.1±4.6 µmol/kg/min in 10 Australian diabetic subjects with significant OSA after 4 months CPAP (31). In another small study of 9 German diabetics, 3 months of CPAP improved insulin sensitivity, but had no significant impact upon HbA1c levels (32). A novel study of 20 diabetics with newly diagnosed OSA (AHI >15) evaluated nocturnal interstitial glucose measurements before and after 3 weeks of CPAP therapy, finding a significant decrease with CPAP [from (122.0±61.7) to (102.9±39.4) mg/dL; P=0.03] despite many of the participants gaining weight between the two studies (33). Unsurprisingly given the short duration of this study, no change was seen in HbA1c levels.
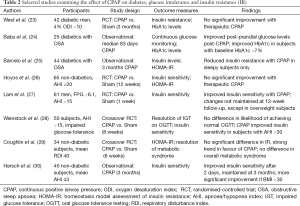
Full table
Conflicting results were produced by two of the more robust studies in this area. Babu et al. evaluated 25 obese diabetic subjects with OSA who were naïve to CPAP treatment (24). HbA1c levels and fasting interstitial glucose were measured before and after an average of 83 days of CPAP use. No significant change was observed in HbA1c levels in the overall cohort, but analyses restricted to subjects with a baseline HbA1c ≥7% or those with objective CPAP usage >4 h/night showed a reduction in HbA1c measurements. Furthermore, the improvement in glycaemic control correlated significantly with the number of nights of CPAP use (r=0.74; P=0.006). In the only randomised controlled trial specifically assessing the influence of CPAP therapy on diabetic control, 42 men with known T2DM and newly diagnosed OSA (ODI >10) were given therapeutic or sham CPAP for 3 months (23). As expected, those in the treatment group had improvements in daytime sleepiness and maintenance of wakefulness test results. However, no improvements in HbA1c levels or measures of insulin sensitivity were observed. Overall CPAP compliance in the treatment arm was sub-optimal (average nightly use 3.6 h), but even with the exclusion of those who were particularly non-compliant, there was no benefit seen in glycaemic control with CPAP. Hence, it remains unproven if CPAP can act as an adjunct in diabetes control in diabetic subjects with sleep disordered breathing.
Association between obstructive sleep apnoea (OSA), insulin resistance (IR) and glucose intolerance
Insulin resistance (IR), glucose intolerance and obstructive sleep apnoea (OSA)
IR among non-diabetic subjects is associated with subsequent increased risk of T2DM and cardiovascular morbidity, and is an independent predictor of overall and cardiac mortality (34). Attempts to evaluate the relationship of OSA with IR are again complicated by the pivotal role played by obesity in driving these disorders, but an expanding evidence base is supportive of the existence of an independent link.
Clinic-based and case control studies from North America, Asia and Europe have suggested that the presence and severity of OSA is independently associated with IR. Among 270 non-diabetic subjects attending a Hong Kong sleep clinic, 68.5% of whom had an AHI ≥5, homeostasis model assessment of insulin resistance (HOMA-IR) was higher in those with OSA and increased with increasing OSA severity (35). This relationship survived adjustment for confounding factors, and was observed in both obese and non-obese subjects. Punjabi et al. studied 150 middle-aged, overweight or mildly obese men, who were free of known cardiometabolic disease (36). An AHI ≥5 was associated with an increased risk of glucose intolerance (OR =2.15; 95% CI, 1.05-4.38) independently of the effect of obesity.
Data focusing on morbidly obese subjects and women have shown similar relationships. Severity of sleep disordered breathing predicted an elevated HOMA-IR in 90 patients recruited from a bariatric clinic (37), while increasing OSA severity was associated with decreased insulin sensitivity in a cohort of 400 non-diabetic Swedish women (11). In the majority of these studies, measures of nocturnal hypoxaemia had the most robust relationship with impaired glycaemic health, suggesting a potential pathophysiological role of IH. Underscoring this, data from 394 participants in the Cleveland Family Study showed the overall burden of nocturnal hypoxaemia (expressed as the cumulative sleep time with SpO2 <90%) to be the key predictor of impaired glucose tolerance (IGT) in OSA patients (38).
There are limited population-level data in this area (Table 1). In a community-based North American study of 2,656 subjects, 8.7% with a respiratory disturbance index (RDI) <5 had IGT on fasting plasma glucose measurement vs. 17.5% with an RDI ≥15, a relationship which persisted after statistical adjustment for confounding variables (adjusted OR =1.46; 95% CI, 1.09-1.97) (10). In an evaluation of the relationship of HbA1c levels with indices of OSA severity in 1,599 subjects without diabetes attending French sleep laboratories (12), multivariate regression analysis showed an association between increased HbA1c levels and OSA severity, most marked in patients with an AHI ≥50 (adjusted OR =2.96; 95% CI, 1.58-5.54). Most recently, a similar analysis of 5,294 non-diabetic participants in the pan-European ESADA study, showed a dose dependent relationship between glycaemic health and severity of sleep disordered breathing, with a significantly higher adjusted HbA1c level in highest AHI quartile (5.50%; 95% CI, 5.46-5.53%) than in the lowest (5.24%; 95% CI, 5.21-5.27%) (13).
Treatment of obstructive sleep apnoea (OSA) and insulin resistance (IR)
The effect of CPAP therapy on insulin sensitivity in non-diabetic subjects remains controversial, with uncontrolled studies suggesting some benefit, but randomised trials providing much more uncertain data (Table 2). Insulin sensitivity improved with two nights of nocturnal CPAP in 40 treatment-naïve, non-diabetic German subjects (30). This effect was maintained at 3 months, but appeared to be largely confined to non-obese patients. A Spanish study of 44 men with newly diagnosed OSA also found treatment with CPAP to be associated with reductions in HOMA-IR and insulin levels, but only in subjects with objective daytime sleepiness at baseline (25).
A randomised, double-blind, crossover trial enrolled 35 British patients free of diabetes, including subjects with known IR (29). Six weeks of CPAP lowered HOMA-IR to a greater degree than sham CPAP, but the difference did not reach statistical significance (Δ=−0.6; 95% CI, −1.3-0.1; P=0.08), whereas daytime sleepiness and blood pressure improved significantly with treatment. Meanwhile, a study of 61 Chinese subjects with an AHI ≥15 randomised to therapeutic vs. sham CPAP for 1 week found insulin sensitivity improved significantly in the treatment group (27). However, a longer term follow-up of the patients who received therapeutic CPAP found no significant change from baseline insulin sensitivity at 3 months, and the authors excluded all subjects with known IR, making its applicability to other OSA cohorts uncertain. Similarly, data from the United States and Australia suggest that CPAP may only have a beneficial effect on glucose tolerance in individuals with severe OSA (28), or on insulin sensitivity after prolonged utilisation (26), respectively.
A number of recent meta-analyses have assessed the effect of CPAP therapy on IR in non-diabetic patients with OSA. These analyses included both uncontrolled studies and randomised-controlled trials (RCTs), but overall did suggest some reduction in HOMA-IR with medium-term use of CPAP, at least amongst compliant patients (39,40). However, there is a clear need for large, well-designed RCTs in this area.
Obstructive sleep apnoea (OSA) and metabolic syndrome (MetS)
While a number of competing, more precise definitions exist (41,42), the MetS is broadly composed of coexistent IR, hypertension, abdominal obesity and dyslipidaemia, and is associated with increased cardiovascular mortality (43). MetS is highly prevalent in OSA cohorts (44), occurring in up to 87% of OSA patients in some series (45). Once again, the confounding effect of visceral adiposity has made it difficult to definitively identify an independent relationship between the two conditions.
There are only a limited number of well-conducted studies examining the effect of CPAP therapy on MetS as an entity. When 34 British subjects with moderate-severe OSA were commenced on 6 weeks of CPAP in a sham-controlled, cross-over fashion, daytime sleepiness and BP improved on CPAP, but the overall prevalence of MetS did not (29). Similarly, in a secondary analysis of a study involving 65 men randomised to therapeutic or sham CPAP for 12 weeks, no significant differences were observed between the two groups in regression or development of MetS (46). A number of uncontrolled studies have suggested that CPAP may be beneficial in this context (47), and CPAP certainly can improve individual component factors of MetS, particularly hypertension (48). However, there is a need for large, prospective RCTs in this field.
In summary, OSA is associated with increased likelihood of T2DM, IR and MetS, and diabetic subjects with severe OSA appear to be less likely to achieve adequate glycaemic control. However, it remains to be established if CPAP therapy can improve metabolic health in pre-diabetic or diabetic subjects.
Potential mechanisms of glucose metabolic dysregulation in obstructive sleep apnoea (OSA)
The pathophysiological mechanisms of alterations in glucose metabolism in OSA are incompletely understood. The process is likely multifactorial and our current concept involves sympathetic nervous system overactivity, systemic and adipose inflammation, oxidative stress and hormonal alterations among the most important pathways. Although the evidence for a causal link remains limited, the major characteristics of OSA, namely sleep fragmentation/deprivation and IH, likely play pivotal roles as triggering factors of the pathophysiology.
Sleep fragmentation/deprivation and glucose metabolic dysfunction
Sleep fragmentation and reduction in total sleep time are characteristic features of OSA. Although this subject is poorly investigated directly in OSA cohorts, one could extrapolate data on sleep fragmentation and reduction in other settings. There is a stream of laboratory and epidemiological data showing an association of these features with T2DM and IR. Several cross-sectional and longitudinal studies, mainly using self-reported sleep duration, suggest that short sleep duration (generally defined as less than 6 h of sleep) is associated with increased risk of T2DM (49-54). In a recent meta-analysis, the relative risk (RR) for the development of T2DM with short sleep was estimated as 1.28 (55). In experimental studies, restricting sleep duration in healthy young subjects leads to alteration in glucose metabolism indicated by a decrease in insulin sensitivity, with subsequent reduced glucose tolerance (56-58).
Independently of reductions in sleep duration, sleep fragmentation with suppression of slow wave sleep also results in impaired insulin sensitivity without compensatory increase in insulin release, as demonstrated in a recent study on healthy volunteers (59). In addition, prospective population-based studies have shown an association between self-reported poor sleep quality and incident T2DM (53,60-62).
The mechanisms by which sleep deprivation and fragmentation contribute to alterations in glucose metabolism are incompletely understood. Sleep deprivation has been found to induce a pro-inflammatory state, with an increase in the release of interleukin (IL)-6 and tumour necrosis factor (TNF)-α and decreased adiponectin levels (63-66)—alterations which have been linked to IR (67). However, negative findings have also been reported, possibly due to considerable variability in baseline levels of these markers and normal circadian fluctuations (68-70). Gender differences have also been observed, and some studies have suggested that sleep restriction was associated with elevation in pro-inflammatory markers in men but not in women (66).
There is also compelling evidence for a role of sympathetic activation—which negatively affects insulin secretion and sensitivity (71,72)—in response to sleep deprivation and fragmentation, reflected in an increase in blood pressure, lower heart rate variability and decreased baroreflex sensitivity (58,73). Some studies also reported increased catecholamine concentration following sleep deprivation (56,57,69). Disturbances in the secretory profiles of the hormones cortisol and growth hormone (GH) may also contribute to the pathogenesis. Cortisol inhibits insulin secretion and elevations of cortisol in response to sleep deprivation and fragmentation have been detected in various studies (56,58,74-76). GH inhibits insulin activity, and usually peaks in the first half of the sleep period. Sleep restriction appears to be associated with a longer elevation of GH secretion during the night, and also a large secretory burst before sleep onset, leading to adverse effects on glucose regulation (77,78).
Several short-term laboratory studies support the assumption that short sleep alters the hormonal regulation of food intake by increasing levels of the appetite-stimulant hormone ghrelin, and by reducing levels of the suppressor leptin, leading to the subjective feeling of hunger with consequent increased caloric intake (79). Furthermore, there is evidence that daytime sleepiness and fatigue as a result of insufficient sleep may lead to reduction in physical activity and subsequent energy expenditure (80).
Collectively, sleep deprivation and fragmentation may contribute to metabolic dysfunction in OSA by various mechanisms. The direct relationships of these pathophysiological triggers in OSA populations, however, remain unknown, and there is a distinct lack of well-designed studies investigating this area.
Intermittent hypoxia (IH) and glucose metabolic dysfunction
IH, characterized by short repetitive cycles of desaturation followed by rapid reoxygenation, is the hallmark feature of OSA and is increasingly recognized as the major pathophysiological trigger for cardiovascular and metabolic disease processes associated with the disease (81). Cell culture and animal models have been developed, allowing the study of IH as a single component of the disease, precisely controlling the triggering events, both in severity and duration and thus, in vitro and animal studies have greatly contributed to our current knowledge. In lean and obese mice, chronic IH exposure contributes to decreased insulin sensitivity, an effect which is partially reversible after cessation of the stimulus (82-85). IH may affect glucose metabolism through systemic effects, including sympathetic excitation, inflammation or oxidative stress and through direct effects on adipose tissue, liver and pancreas.
Intermittent hypoxia (IH), sympathetic activation, systemic inflammation and oxidative stress
IH has been demonstrated to trigger multiple pathways potentially involved in the pathogenesis of cardiovascular and metabolic diseases (81). Sympathetic excitation in response to IH has been extensively studied in humans and rodents, and is widely believed to contribute to arterial systemic hypertension and other cardiovascular consequences (86). In addition, catecholamines are known to decrease insulin sensitivity and to reduce insulin-mediated glucose uptake (71,72). Several studies have demonstrated increased blood pressure and an associated rise in sympathetic activity in OSA patients versus matched controls, with improvement following short-term CPAP therapy (87-90). IH rodent models reproduce these changes, with blood pressure increases due to IH preventable by pharmacological and surgical blockage of the sympathetic nervous system (91,92). A recent study investigated the effect of adrenal medullectomy on glucose metabolism in mice treated with IH, and although surgery led to improved insulin secretion, IH-induced hyperglycaemia and IR remained unaffected, supporting the need for further studies on this subject (93).
OSA-related IH has generally been associated with increased production of reactive oxygen species (ROS) and thus is thought to promote oxidative stress, which may contribute to alteration in glucose metabolism (94). Nonetheless, supporting data are sparse and results have been inconsistent. Jelic et al. harvested venous endothelial cells from OSA patients and matched controls, detecting higher levels of the oxidative stress marker nitrotyrosine in OSA subjects, with a significant fall following CPAP therapy (95). Studies in rats have demonstrated that IH leads to increased lipid peroxidation which correlated with left ventricular dysfunction (96). However, large studies have failed to show a beneficial effect of antioxidant therapy on cardiovascular diseases, and oxidative stress could potentially be a consequence rather than a cause of vascular inflammation and subsequent atherosclerosis (97).
Vascular and systemic inflammation are central in the pathogenesis of OSA-associated cardiometabolic processes and there is overwhelming support from human and animals studies of the key role of IH in driving inflammatory responses (98-100). IH activates the transcription factor nuclear factor-kappa B (NF-κB) (101-103). NF-κB is the master regulator of an inflammatory response, and numerous inflammatory, pro-atherogenic genes, such as TNF-α, IL-8 or intercellular adhesion molecule 1 (ICAM-1), are under its control. NF-κB activation has been demonstrated in cardiovascular tissue from mice treated with IH, and in cultured monocytes from OSA patients (101,103). Using a cell culture model of IH, our group demonstrated a preferential activation of NF-κB-dependent pro-inflammatory pathways by IH over adaptive, hypoxia-inducible factor 1 (HIF-1) mediated pathways, which is in contrast to sustained hypoxia where adaptive pathways predominate (102). Systemic and vascular levels of NF-κB-dependent pro-inflammatory mediators have been found to be increased in OSA patients vs. controls, potentially contributing to endothelial dysfunction, and CPAP therapy has a beneficial effect on these effects (100,104,105).
Effect of IH on adipose tissue
There is increasing evidence for an interaction between OSA and obesity in the development of cardiovascular and metabolic diseases. Obesity is strongly associated with OSA—the prevalence of OSA in obese subjects exceeds 30% and at least 60% of OSA patients are obese (106). Obesity exerts many of its cardiovascular and metabolic complications through the action of the white adipose tissue (WAT). WAT has been identified as a highly active endocrine organ secreting multiple proteins—termed adipokines—which contribute to many pathophysiological processes (107). Metabolically dysfunctional adipose tissue is characterized by infiltration of macrophages and other immune cells including T-lymphocytes and mast cells. In addition, macrophages associated with obesity are polarized to a M1 or “classically activated” phenotype, and these M1 macrophages produce pro-inflammatory cytokines such as IL-6 and TNF-α, express inducible nitric oxide synthase (iNOS) and promote obesity-induced IR. In contrast, in lean subjects macrophages predominantly show an alternative, M2-pattern of expression with upregulation of anti-inflammatory factors such as IL-10 or adiponectin, and downregulation of pro-inflammatory cytokines, and thus are associated with tissue repair and resolution of inflammation (108).
There is emerging evidence that in obesity, where there is excess of adipose tissue, hypoxia is a key factor in modulating the pro-inflammatory response of WAT (109). As IH represents a stronger inflammatory stimulus than sustained hypoxia, this process may be potentiated by IH associated with OSA. Various studies have demonstrated that WAT in obese humans or rodents is more hypoxic than in lean controls (110,111). Given the technical difficulties in the direct measurement of rapid fluctuations in oxygen concentrations in tissue there are currently no data on the potential additive effect of IH in OSA subjects. Reinke et al. investigated the oxygen profile in response to IH in a mouse model and suggested that the oxygen fluctuations of IH are attenuated in adipose tissue (112). It is unknown, however, how this potentially relates to human adiposity and it is likely that there will be significant local differences within tissue depending on the relative distance to the circulatory system.
The master transcriptional regulator in response to hypoxia is HIF-1, which regulates transcription of numerous genes affecting various processes such as angiogenesis, metabolism, vascular tone and cell survival (113). It has recently been shown that HIF-1 activation occurs at the onset of obesity as a response to relative tissue hypoxia leading to adipose tissue inflammation and metabolic dysfunction (114); importantly genetic or pharmacological inhibition of HIF-1 proved protective in this setting. Meanwhile, hypoxia has previously been shown to inhibit insulin signalling and glucose transport in human and murine adipocytes in a HIF-1 dependent manner (115). Adipose HIF-1 activation in response to IH has also been recently demonstrated in ApoE-deficient lean mice, and this event was associated with accelerated atherosclerosis (116). Using the same mouse strain, the hypoxia research group of the University of Grenoble, France, recently demonstrated increased macrophage infiltration of adipose tissue and increased expression of monocyte chemoattractant protein (MCP)-1, abnormalities which were associated with reduced insulin sensitivity and more advanced atherosclerotic lesions (117). However, in morbidly obese patients, IH does not appear to worsen macrophage accumulation in omental and subcutaneous fat depots (118), but there are no data outside the morbidly obese population. In support of a mechanistic link between IH and adipose tissue, Gharib et al. identified a profound effect of IH on gene expression in WAT mapping to numerous processes, including metabolism and oxidative stress responses (119). As a potential explanation, primary human adipocytes exposed to IH in vitro are significantly more sensitive to this pro-inflammatory stimulus than other primary cells, with the downstream consequence of increased activation of the NF-κB pathway and resultant expression of multiple inflammatory mediators known to play key roles in glucose metabolic dysfunction (120).
In summary, rapidly increasing evidence points to an additive effect of IH on adipose tissue inflammation, but further translational studies are urgently required to determine its detailed role.
Effect of IH on liver damage
Non-alcoholic fatty liver disease (NAFLD) is a spectrum of liver disease characterized by the presence of ectopic fat in the liver, steatosis, which cannot be explained by alcohol consumption. It ranges from simple steatosis to non-alcoholic steatohepatits (NASH), that can progress to liver cirrhosis and is associated with the development of hepatocellular carcinoma (121). NAFLD is strongly associated with obesity, IR/T2DM and MetS (122). The relationship of NAFLD and IR is bi-directional, suggesting a self-perpetuating vicious cycle. Aron-Wisnewsky et al. systematically studied liver histology and overnight oximetry in morbidly obese patients undergoing bariatric surgery, and detected that the severity of IH—as indicated by the ODI—was associated with more severe liver injury, consistent with results of previous studies on OSA populations (118). Animal studies strongly support the detrimental role of IH on NAFLD progression, particularly evident in obese mice (82,123). The mechanisms underlying IH as “second hit” stimulus for NAFLD progression are largely unexplored and warrant further detailed studies. Furthermore, the role of CPAP therapy in potentially preventing NAFLD progression will need to be determined.
Effect of IH on pancreatic B-cell function
B-cell dysfunction in response to IH is suspected based on the frequent observation that decreases in insulin sensitivity are often not matched by compensatory increase in insulin secretion. Louis et al. exposed a group of healthy volunteers to 8 h of IH or intermittent air in a randomized crossover design study, and despite worsening insulin sensitivity with IH, pancreatic insulin secretion was similar between both treatments (124). These results have subsequently been reproduced in mice and rats exposed to IH (83,84,125,126) and as a potential explanation, Polak et al. detected increased protein levels of the oxidative stress marker thiobarbituric acid reactive substances in pancreas isolated from mice treated with IH vs. intermittent air (84). The detailed mechanisms including the role of oxidative stress in this process, nonetheless, warrant further detailed investigation.
Conclusions and future directions
Evidence for an association of OSA and glucose metabolic dysfunction is rapidly growing. However, much of this evidence is derived from cross-sectional analyses and there is a clear need for large, well-designed, prospective studies. The lack of benefit with CPAP therapy in numerous studies demands a greater insight into the interaction of OSA and other contributing factors, in particular obesity, in the pathogenesis of alterations in glucose metabolism. IH and sleep deprivation/fragmentation likely play pivotal roles in the pathogenesis of glucose metabolic dysfunction in OSA, potentially contributing to multiple pathways likely synergistically with obesity (Figure 1). To further define the mechanisms underlying these processes, clinical and translational studies are urgently required.
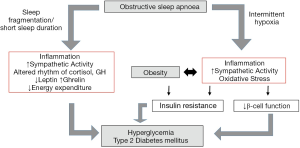
Acknowledgements
Funding: This work was supported by the Health Research Board of Ireland (SR).
Footnote
Conflicts of Interest: The authors have no conflicts of interest to declare.
References
- Peppard PE, Young T, Barnet JH, et al. Increased Prevalence of Sleep-Disordered Breathing in Adults. Am J Epidemiol 2013;177:1006-14. [PubMed]
- McNicholas WT, Bonsigore MR. Management Committee of EU COST ACTION B26. Sleep apnoea as an independent risk factor for cardiovascular disease: current evidence, basic mechanisms and research priorities. Eur Respir J 2007;29:156-78. [PubMed]
- West SD, Nicoll DJ, Stradling JR. Prevalence of obstructive sleep apnoea in men with type 2 diabetes. Thorax 2006;61:945-50. [PubMed]
- Foster GD, Sanders MH, Millman R, et al. Obstructive sleep apnea among obese patients with type 2 diabetes. Diabetes Care 2009;32:1017-9. [PubMed]
- Reichmuth KJ, Austin D, Skatrud JB, et al. Association of sleep apnea and type II diabetes: a population-based study. Am J Respir Crit Care Med 2005;172:1590-5. [PubMed]
- Ronksley PE, Hemmelgarn BR, Heitman SJ, et al. Obstructive sleep apnoea is associated with diabetes in sleepy subjects. Thorax 2009;64:834-9. [PubMed]
- Botros N, Concato J, Mohsenin V, et al. Obstructive sleep apnea as a risk factor for type 2 diabetes. Am J Med 2009;122:1122-7. [PubMed]
- Kent BD, Grote L, Ryan S, et al. Diabetes Mellitus Prevalence and Control in Sleep-Disordered Breathing: The European Sleep Apnea Cohort (ESADA) Study. Chest 2014;146:982-90. [PubMed]
- Kendzerska T, Gershon AS, Hawker G, et al. Obstructive sleep apnea and incident diabetes. A historical cohort study. Am J Respir Crit Care Med 2014;190:218-25. [PubMed]
- Punjabi NM, Shahar E, Redline S, et al. Sleep-disordered breathing, glucose intolerance, and insulin resistance: the Sleep Heart Health Study. Am J Epidemiol 2004;160:521-30. [PubMed]
- Theorell-Haglöw J, Berne C, Janson C, et al. Obstructive sleep apnoea is associated with decreased insulin sensitivity in females. Eur Respir J 2008;31:1054-60. [PubMed]
- Priou P, Le Vaillant M, Meslier N, et al. Independent association between obstructive sleep apnea severity and glycated hemoglobin in adults without diabetes. Diabetes Care 2012;35:1902-6. [PubMed]
- Kent BD, Grote L, Bonsignore MR, et al. Sleep apnoea severity independently predicts glycaemic health in nondiabetic subjects: the ESADA study. Eur Respir J 2014;44:130-9. [PubMed]
- Gottlieb DJ, Yenokyan G, Newman AB, et al. Prospective study of obstructive sleep apnea and incident coronary heart disease and heart failure: the sleep heart health study. Circulation 2010;122:352-60. [PubMed]
- American Diabetes Association. Diagnosis and classification of diabetes mellitus. Diabetes Care 2011;34 Suppl 1:S62-9. [PubMed]
- Fitzgerald DB, Kent BD, Garvey JF, et al. Screening for diabetes mellitus in patients with OSAS: a case for glycosylated haemoglobin. Eur Respir J 2012;40:273-4. [PubMed]
- Meslier N, Gagnadoux F, Giraud P, et al. Impaired glucose-insulin metabolism in males with obstructive sleep apnoea syndrome. Eur Respir J 2003;22:156-60. [PubMed]
- Tamura A, Kawano Y, Watanabe T, et al. Relationship between the severity of obstructive sleep apnea and impaired glucose metabolism in patients with obstructive sleep apnea. Respir Med 2008;102:1412-6. [PubMed]
- Elmasry A, Janson C, Lindberg E, et al. The role of habitual snoring and obesity in the development of diabetes: a 10-year follow-up study in a male population. J Intern Med 2000;248:13-20. [PubMed]
- Al-Delaimy WK, Manson JE, Willett WC, et al. Snoring as a risk factor for type II diabetes mellitus: a prospective study. Am J Epidemiol 2002;155:387-93. [PubMed]
- Aronsohn RS, Whitmore H, Van Cauter E, et al. Impact of untreated obstructive sleep apnea on glucose control in type 2 diabetes. Am J Respir Crit Care Med 2010;181:507-13. [PubMed]
- American Diabetes Association. Standards of medical care in diabetes--2013. Diabetes Care 2013;36 Suppl 1:S11-66. [PubMed]
- West SD, Nicoll DJ, Wallace TM, et al. Effect of CPAP on insulin resistance and HbA1c in men with obstructive sleep apnoea and type 2 diabetes. Thorax 2007;62:969-74. [PubMed]
- Babu AR, Herdegen J, Fogelfeld L, et al. Type 2 diabetes, glycemic control, and continuous positive airway pressure in obstructive sleep apnea. Arch Intern Med 2005;165:447-52. [PubMed]
- Barceló A, Barbé F, de la Peña M, et al. Insulin resistance and daytime sleepiness in patients with sleep apnoea. Thorax 2008;63:946-50. [PubMed]
- Hoyos CM, Killick R, Yee BJ, et al. Cardiometabolic changes after continuous positive airway pressure for obstructive sleep apnoea: a randomised sham-controlled study. Thorax 2012;67:1081-9. [PubMed]
- Lam JC, Lam B, Yao TJ, et al. A randomised controlled trial of nasal continuous positive airway pressure on insulin sensitivity in obstructive sleep apnoea. Eur Respir J 2010;35:138-45. [PubMed]
- Weinstock TG, Wang X, Rueschman M, et al. A controlled trial of CPAP therapy on metabolic control in individuals with impaired glucose tolerance and sleep apnea. Sleep 2012;35:617-625B. [PubMed]
- Coughlin SR, Mawdsley L, Mugarza JA, et al. Cardiovascular and metabolic effects of CPAP in obese males with OSA. Eur Respir J 2007;29:720-7. [PubMed]
- Harsch IA, Schahin SP, Radespiel-Troger M, et al. Continuous positive airway pressure treatment rapidly improves insulin sensitivity in patients with obstructive sleep apnea syndrome. Am J Respir Crit Care Med 2004;169:156-62. [PubMed]
- Brooks B, Cistulli PA, Borkman M, et al. Obstructive sleep apnea in obese noninsulin-dependent diabetic patients: effect of continuous positive airway pressure treatment on insulin responsiveness. J Clin Endocrinol Metab 1994;79:1681-5. [PubMed]
- Harsch IA, Schahin SP, Brückner K, et al. The effect of continuous positive airway pressure treatment on insulin sensitivity in patients with obstructive sleep apnoea syndrome and type 2 diabetes. Respiration 2004;71:252-9. [PubMed]
- Dawson A, Abel SL, Loving RT, et al. CPAP therapy of obstructive sleep apnea in type 2 diabetics improves glycemic control during sleep. J Clin Sleep Med 2008;4:538-42. [PubMed]
- Selvin E, Steffes MW, Zhu H, et al. Glycated hemoglobin, diabetes, and cardiovascular risk in nondiabetic adults. N Engl J Med 2010;362:800-11. [PubMed]
- Ip MS, Lam B, Ng MM, et al. Obstructive sleep apnea is independently associated with insulin resistance. Am J Respir Crit Care Med 2002;165:670-6. [PubMed]
- Punjabi NM, Sorkin JD, Katzel LI, et al. Sleep-disordered breathing and insulin resistance in middle-aged and overweight men. Am J Respir Crit Care Med 2002;165:677-82. [PubMed]
- Polotsky VY, Patil SP, Savransky V, et al. Obstructive sleep apnea, insulin resistance, and steatohepatitis in severe obesity. Am J Respir Crit Care Med 2009;179:228-34. [PubMed]
- Sulit L, Storfer-Isser A, Kirchner HL, et al. Differences in polysomnography predictors for hypertension and impaired glucose tolerance. Sleep 2006;29:777-83. [PubMed]
- Yang D, Liu Z, Yang H. The impact of effective continuous positive airway pressure on homeostasis model assessment insulin resistance in non-diabetic patients with moderate to severe obstructive sleep apnea. Diabetes Metab Res Rev 2012;28:499-504. [PubMed]
- Iftikhar IH, Khan MF, Das A, et al. Meta-analysis: continuous positive airway pressure improves insulin resistance in patients with sleep apnea without diabetes. Ann Am Thorac Soc 2013;10:115-20. [PubMed]
- Grundy SM, Cleeman JI, Daniels SR, et al. Diagnosis and management of the metabolic syndrome: an American Heart Association/National Heart, Lung, and Blood Institute Scientific Statement. Circulation 2005;112:2735-52. [PubMed]
- Alberti KG, Zimmet P, Shaw J, et al. The metabolic syndrome--a new worldwide definition. Lancet 2005;366:1059-62. [PubMed]
- Ho JS, Cannaday JJ, Barlow CE, et al. Relation of the number of metabolic syndrome risk factors with all-cause and cardiovascular mortality. Am J Cardiol 2008;102:689-92. [PubMed]
- Gasa M, Salord N, Fortuna AM, et al. Obstructive sleep apnoea and metabolic impairment in severe obesity. Eur Respir J 2011;38:1089-97. [PubMed]
- Coughlin SR, Mawdsley L, Mugarza JA, et al. Obstructive sleep apnoea is independently associated with an increased prevalence of metabolic syndrome. Eur Heart J 2004;25:735-41. [PubMed]
- Hoyos CM, Sullivan DR, Liu PY. Effect of CPAP on the metabolic syndrome: a randomised sham-controlled study. Thorax 2013;68:588-9. [PubMed]
- Dorkova Z, Petrasova D, Molcanyiova A, et al. Effects of continuous positive airway pressure on cardiovascular risk profile in patients with severe obstructive sleep apnea and metabolic syndrome. Chest 2008;134:686-92. [PubMed]
- Fava C, Dorigoni S, Dalle Vedove F, et al. Effect of CPAP on blood pressure in patients with OSA/hypopnea a systematic review and meta-analysis. Chest 2014;145:762-71. [PubMed]
- Ayas NT, White DP, Al-Delaimy WK, et al. A prospective study of self-reported sleep duration and incident diabetes in women. Diabetes Care 2003;26:380-4. [PubMed]
- Chaput JP, Despres JP, Bouchard C, et al. Sleep duration as a risk factor for the development of type 2 diabetes or impaired glucose tolerance: analyses of the Quebec Family Study. Sleep Med 2009;10:919-24. [PubMed]
- Gottlieb DJ, Punjabi NM, Newman AB, et al. Association of sleep time with diabetes mellitus and impaired glucose tolerance. Arch Intern Med 2005;165:863-7. [PubMed]
- Mallon L, Broman JE, Hetta J. High incidence of diabetes in men with sleep complaints or short sleep duration: a 12-year follow-up study of a middle-aged population. Diabetes Care 2005;28:2762-7. [PubMed]
- Nilsson PM, Roost M, Engstrom G, et al. Incidence of diabetes in middle-aged men is related to sleep disturbances. Diabetes Care 2004;27:2464-9. [PubMed]
- Yaggi HK, Araujo AB, McKinlay JB. Sleep duration as a risk factor for the development of type 2 diabetes. Diabetes Care 2006;29:657-61. [PubMed]
- Cappuccio FP, D'Elia L, Strazzullo P, et al. Quantity and quality of sleep and incidence of type 2 diabetes: a systematic review and meta-analysis. Diabetes Care 2010;33:414-20. [PubMed]
- Buxton OM, Pavlova M, Reid EW, et al. Sleep restriction for 1 week reduces insulin sensitivity in healthy men. Diabetes 2010;59:2126-33. [PubMed]
- Nedeltcheva AV, Kessler L, Imperial J, et al. Exposure to recurrent sleep restriction in the setting of high caloric intake and physical inactivity results in increased insulin resistance and reduced glucose tolerance. J Clin Endocrinol Metab 2009;94:3242-50. [PubMed]
- Spiegel K, Leproult R, Van Cauter E. Impact of sleep debt on metabolic and endocrine function. Lancet 1999;354:1435-9. [PubMed]
- Tasali E, Leproult R, Ehrmann DA, et al. Slow-wave sleep and the risk of type 2 diabetes in humans. Proc Natl Acad Sci U S A 2008;105:1044-9. [PubMed]
- Hayashino Y, Fukuhara S, Suzukamo Y, et al. Relation between sleep quality and quantity, quality of life, and risk of developing diabetes in healthy workers in Japan: the High-risk and Population Strategy for Occupational Health Promotion (HIPOP-OHP) Study. BMC Public Health 2007;7:129. [PubMed]
- Kawakami N, Takatsuka N, Shimizu H. Sleep disturbance and onset of type 2 diabetes. Diabetes Care 2004;27:282-3. [PubMed]
- Meisinger C, Heier M, Loewel H, et al. Sleep disturbance as a predictor of type 2 diabetes mellitus in men and women from the general population. Diabetologia 2005;48:235-41. [PubMed]
- Irwin MR, Wang M, Campomayor CO, et al. Sleep deprivation and activation of morning levels of cellular and genomic markers of inflammation. Arch Intern Med 2006;166:1756-62. [PubMed]
- Maeda N, Shimomura I, Kishida K, et al. Diet-induced insulin resistance in mice lacking adiponectin/ACRP30. Nat Med 2002;8:731-7. [PubMed]
- Shearer WT, Reuben JM, Mullington JM, et al. Soluble TNF-alpha receptor 1 and IL-6 plasma levels in humans subjected to the sleep deprivation model of spaceflight. J Allergy Clin Immunol 2001;107:165-70. [PubMed]
- Vgontzas AN, Zoumakis E, Bixler EO, et al. Adverse effects of modest sleep restriction on sleepiness, performance, and inflammatory cytokines. J Clin Endocrinol Metab 2004;89:2119-26. [PubMed]
- Wieser V, Moschen AR, Tilg H. Inflammation, cytokines and insulin resistance: a clinical perspective. Arch Immunol Ther Exp (Warsz) 2013;61:119-25. [PubMed]
- Bauer J, Hohagen F, Ebert T, et al. Interleukin-6 serum levels in healthy persons correspond to the sleep-wake cycle. Clin Investig 1994;72:315. [PubMed]
- Mullington JM, Simpson NS, Meier-Ewert HK, et al. Sleep loss and inflammation. Best Pract Res Clin Endocrinol Metab 2010;24:775-84. [PubMed]
- Vgontzas AN, Zoumakis M, Papanicolaou DA, et al. Chronic insomnia is associated with a shift of interleukin-6 and tumor necrosis factor secretion from nighttime to daytime. Metabolism 2002;51:887-92. [PubMed]
- Deibert DC, DeFronzo RA. Epinephrine-induced insulin resistance in man. J Clin Invest 1980;65:717-21. [PubMed]
- Dungan KM, Braithwaite SS, Preiser JC. Stress hyperglycaemia. Lancet 2009;373:1798-807. [PubMed]
- Stamatakis KA, Punjabi NM. Effects of sleep fragmentation on glucose metabolism in normal subjects. Chest 2010;137:95-101. [PubMed]
- Leproult R, Copinschi G, Buxton O, et al. Sleep loss results in an elevation of cortisol levels the next evening. Sleep 1997;20:865-70. [PubMed]
- Späth-Schwalbe E, Uthgenannt D, Voget G, et al. Corticotropin-releasing hormone-induced adrenocorticotropin and cortisol secretion depends on sleep and wakefulness. J Clin Endocrinol Metab 1993;77:1170-3. [PubMed]
- Weitzman ED, Zimmerman JC, Czeisler CA, et al. Cortisol secretion is inhibited during sleep in normal man. J Clin Endocrinol Metab 1983;56:352-8. [PubMed]
- Leproult R, Van Cauter E. Role of sleep and sleep loss in hormonal release and metabolism. Endocr Dev 2010;17:11-21. [PubMed]
- Mullington JM, Haack M, Toth M, et al. Cardiovascular, inflammatory, and metabolic consequences of sleep deprivation. Prog Cardiovasc Dis 2009;51:294-302. [PubMed]
- Spiegel K, Tasali E, Penev P, et al. Brief communication: Sleep curtailment in healthy young men is associated with decreased leptin levels, elevated ghrelin levels, and increased hunger and appetite. Ann Intern Med 2004;141:846-50. [PubMed]
- O'Driscoll DM, Turton AR, Copland JM, et al. Energy expenditure in obstructive sleep apnea: validation of a multiple physiological sensor for determination of sleep and wake. Sleep Breath 2013;17:139-46. [PubMed]
- Lévy P, Ryan S, Oldenburg O, et al. Sleep apnoea and the heart. Eur Respir Rev 2013;22:333-52. [PubMed]
- Drager LF, Li J, Reinke C, et al. Intermittent hypoxia exacerbates metabolic effects of diet-induced obesity. Obesity (Silver Spring) 2011;19:2167-74. [PubMed]
- Lee EJ, Alonso LC, Stefanovski D, et al. Time-dependent changes in glucose and insulin regulation during intermittent hypoxia and continuous hypoxia. Eur J Appl Physiol 2013;113:467-78. [PubMed]
- Polak J, Shimoda LA, Drager LF, et al. Intermittent hypoxia impairs glucose homeostasis in C57BL6/J mice: partial improvement with cessation of the exposure. Sleep 2013;36:1483-90; 1490A-1490B.
- Polotsky VY, Li J, Punjabi NM, et al. Intermittent hypoxia increases insulin resistance in genetically obese mice. J Physiol 2003;552:253-64. [PubMed]
- Fletcher EC. Sympathetic over activity in the etiology of hypertension of obstructive sleep apnea. Sleep 2003;26:15-9. [PubMed]
- Carlson JT, Hedner J, Elam M, et al. Augmented resting sympathetic activity in awake patients with obstructive sleep apnea. Chest 1993;103:1763-8. [PubMed]
- Narkiewicz K, Kato M, Phillips BG, et al. Nocturnal continuous positive airway pressure decreases daytime sympathetic traffic in obstructive sleep apnea. Circulation 1999;100:2332-5. [PubMed]
- Somers VK, Dyken ME, Clary MP, et al. Sympathetic neural mechanisms in obstructive sleep apnea. J Clin Invest 1995;96:1897-904. [PubMed]
- Ziegler MG, Mills PJ, Loredo JS, et al. Effect of continuous positive airway pressure and placebo treatment on sympathetic nervous activity in patients with obstructive sleep apnea. Chest 2001;120:887-93. [PubMed]
- Bao G, Metreveli N, Li R, et al. Blood pressure response to chronic episodic hypoxia: role of the sympathetic nervous system. J Appl Physiol 1997;83:95-101. [PubMed]
- Fletcher EC, Lesske J, Culman J, et al. Sympathetic denervation blocks blood pressure elevation in episodic hypoxia. Hypertension 1992;20:612-9. [PubMed]
- Shin MK, Han W, Bevans-Fonti S, et al. The effect of adrenal medullectomy on metabolic responses to chronic intermittent hypoxia. Respir Physiol Neurobiol 2014;203:60-7. [PubMed]
- Henriksen EJ, Diamond-Stanic MK, Marchionne EM. Oxidative stress and the etiology of insulin resistance and type 2 diabetes. Free Radic Biol Med 2011;51:993-9. [PubMed]
- Jelic S, Padeletti M, Kawut SM, et al. Inflammation, oxidative stress, and repair capacity of the vascular endothelium in obstructive sleep apnea. Circulation 2008;117:2270-8. [PubMed]
- Chen L, Einbinder E, Zhang Q, et al. Oxidative stress and left ventricular function with chronic intermittent hypoxia in rats. Am J Respir Crit Care Med 2005;172:915-20. [PubMed]
- Lonn E, Bosch J, Yusuf S, et al. Effects of long-term vitamin E supplementation on cardiovascular events and cancer: a randomized controlled trial. JAMA 2005;293:1338-47. [PubMed]
- Arnaud C, Poulain L, Levy P, et al. Inflammation contributes to the atherogenic role of intermittent hypoxia in apolipoprotein-E knock out mice. Atherosclerosis 2011;219:425-31. [PubMed]
- Dyugovskaya L, Lavie P, Lavie L. Phenotypic and functional characterization of blood gammadelta T cells in sleep apnea. Am J Respir Crit Care Med 2003;168:242-9. [PubMed]
- Ryan S, Taylor CT, McNicholas WT. Systemic inflammation: a key factor in the pathogenesis of cardiovascular complications in obstructive sleep apnoea syndrome? Thorax 2009;64:631-6. [PubMed]
- Greenberg H, Ye X, Wilson D, et al. Chronic intermittent hypoxia activates nuclear factor-kappaB in cardiovascular tissues in vivo. Biochem Biophys Res Commun 2006;343:591-6. [PubMed]
- Ryan S, Taylor CT, McNicholas WT. Selective activation of inflammatory pathways by intermittent hypoxia in obstructive sleep apnea syndrome. Circulation 2005;112:2660-7. [PubMed]
- Yamauchi M, Tamaki S, Tomoda K, et al. Evidence for activation of nuclear factor kappaB in obstructive sleep apnea. Sleep Breath 2006;10:189-93. [PubMed]
- Ohga E, Nagase T, Tomita T, et al. Increased levels of circulating ICAM-1, VCAM-1, and L-selectin in obstructive sleep apnea syndrome. J Appl Physiol 1999;87:10-4. [PubMed]
- Ryan S, Taylor CT, McNicholas WT. Predictors of elevated nuclear factor-kappaB-dependent genes in obstructive sleep apnea syndrome. Am J Respir Crit Care Med 2006;174:824-30. [PubMed]
- Peppard PE, Young T, Palta M, et al. Longitudinal study of moderate weight change and sleep-disordered breathing. JAMA 2000;284:3015-21. [PubMed]
- Trayhurn P, Beattie JH. Physiological role of adipose tissue: white adipose tissue as an endocrine and secretory organ. Proc Nutr Soc 2001;60:329-39. [PubMed]
- Ouchi N, Parker JL, Lugus JJ, et al. Adipokines in inflammation and metabolic disease. Nat Rev Immunol. 2011;11:85-97. [PubMed]
- Trayhurn P, Wang B, Wood IS. Hypoxia and the endocrine and signalling role of white adipose tissue. Arch Physiol Biochem 2008;114:267-76. [PubMed]
- Pasarica M, Sereda OR, Redman LM, et al. Reduced adipose tissue oxygenation in human obesity: evidence for rarefaction, macrophage chemotaxis, and inflammation without an angiogenic response. Diabetes 2009;58:718-25. [PubMed]
- Ye J, Gao Z, Yin J, et al. Hypoxia is a potential risk factor for chronic inflammation and adiponectin reduction in adipose tissue of ob/ob and dietary obese mice. Am J Physiol Endocrinol Metab 2007;293:E1118-28. [PubMed]
- Reinke C, Bevans-Fonti S, Drager LF, et al. Effects of different acute hypoxic regimens on tissue oxygen profiles and metabolic outcomes. J Appl Physiol (1985) 2011;111:881-90. [PubMed]
- Huang LE, Bunn HF. Hypoxia-inducible factor and its biomedical relevance. J Biol Chem 2003;278:19575-8. [PubMed]
- Lee YS, Kim JW, Osborne O, et al. Increased adipocyte O2 consumption triggers HIF-1α, causing inflammation and insulin resistance in obesity. Cell 2014;157:1339-52. [PubMed]
- Regazzetti C, Peraldi P, Gremeaux T, et al. Hypoxia decreases insulin signaling pathways in adipocytes. Diabetes 2009;58:95-103. [PubMed]
- Drager LF, Yao Q, Hernandez KL, et al. Chronic intermittent hypoxia induces atherosclerosis via activation of adipose angiopoietin-like 4. Am J Respir Crit Care Med 2013;188:240-8. [PubMed]
- Poulain L, Thomas A, Rieusset J, et al. Visceral white fat remodelling contributes to intermittent hypoxia-induced atherogenesis. Eur Respir J 2014;43:513-22. [PubMed]
- Aron-Wisnewsky J, Minville C, Tordjman J, et al. Chronic intermittent hypoxia is a major trigger for non-alcoholic fatty liver disease in morbid obese. J Hepatol 2012;56:225-33. [PubMed]
- Gharib SA, Khalyfa A, Abdelkarim A, et al. Intermittent hypoxia activates temporally coordinated transcriptional programs in visceral adipose tissue. J Mol Med (Berl) 2012;90:435-45. [PubMed]
- Taylor CT, Kent BD, Crinion SJ, et al. Human adipocytes are highly sensitive to intermittent hypoxia induced NF-kappaB activity and subsequent inflammatory gene expression. Biochem Biophys Res Commun 2014;447:660-5. [PubMed]
- Fassio E, Alvarez E, Dominguez N, et al. Natural history of nonalcoholic steatohepatitis: a longitudinal study of repeat liver biopsies. Hepatology 2004;40:820-6. [PubMed]
- Perry RJ, Samuel VT, Petersen KF, et al. The role of hepatic lipids in hepatic insulin resistance and type 2 diabetes. Nature 2014;510:84-91. [PubMed]
- Savransky V, Bevans S, Nanayakkara A, et al. Chronic intermittent hypoxia causes hepatitis in a mouse model of diet-induced fatty liver. Am J Physiol Gastrointest Liver Physiol 2007;293:G871-7. [PubMed]
- Louis M, Punjabi NM. Effects of acute intermittent hypoxia on glucose metabolism in awake healthy volunteers. J Appl Physiol (1985) 2009;106:1538-44. [PubMed]
- Fenik VB, Singletary T, Branconi JL, et al. Glucoregulatory consequences and cardiorespiratory parameters in rats exposed to chronic-intermittent hypoxia: effects of the duration of exposure and losartan. Front Neurol 2012;3:51. [PubMed]
- Sherwani SI, Aldana C, Usmani S, et al. Intermittent hypoxia exacerbates pancreatic beta-cell dysfunction in a mouse model of diabetes mellitus. Sleep 2013;36:1849-58. [PubMed]