Investigating the multitarget pharmacological mechanism of Rhodiola wallichiana var. cholaensis acting on angina pectoris using combined network pharmacology and molecular docking
Highlight box
Key findings
• The results of this study verify and predict the molecular mechanism of Rhodiola wallichiana var. cholaensis (RW) in angina pectoris (AP) at the system level, which may provide enlightenment for the mechanism of RW and other anti-AP Chinese medicines and promote the application of RW in the treatment of AP.
What is known and what is new?
• RW is a traditional Chinese medicine that activates blood circulation and removes blood stasis, which can effectively relieve patients’ blood circulation disorders and improve the protective effect against cardiovascular diseases.
• We found that these hub targets (VEGFA, GAPDH, TP53, AKT1, CASP3, STAT3, TNF, MAPK1 and JUN) ameliorated AP by participating in protecting the vascular endothelium, regulating glucose metabolism, regulating cellular processes, regulating the inflammatory response, and participating in cell signal transduction, among other processes.
• What is the implication, and what should change now?
The clinical application of RW in cardiovascular diseases has accurate efficacy, high safety, few adverse reactions, and can achieve rapid and effective treatment purposes. It is one of the ideal choices for the treatment of cardiovascular diseases.
Introduction
Angina pectoris (AP) is a clinical syndrome with episodes of chest pain as the main symptoms due to temporary hypoxia and ischemia of the heart (1). The incidence of AP in China is about 3.6%, and the trend is increasing year by year (2). It is well known that AP is a typical symptom of myocardial ischemia. Therefore, conventional medicines for the treatment of AP, such as nitrates, beta-blockers, calcium channel blockers, are mainly used to alleviate the symptoms of AP (3).
Traditional Chinese medicine (TCM) is an integrated system of medicine with a history of thousands of years. It has potential application value in clinical practice (4). Rhodiola wallichiana var. cholaensis (RW) is a common species of the genus Rhodiola, which is one of the most popular medicinal plants in Asia (5). Clinical studies have shown that RW preparations can reduce the symptoms of AP, heart failure and other heart diseases, have antioxidant and anti-inflammatory effects, and reduce hypoxia-induced cellular oxidative stress (6,7). Moreover, modern pharmacological researches have revealed multiple bioactivities of RW plants, such as antioxidative (8,9), immunomodulatory (10), anti-Inflammatory (11), antidiabetic (12,13), antihypertensive and neuroprotective (14,15) antistress and antidepressant (16-18), anti-altitude sickness (19,20), antifatigue (21,22), and anticancer (23,24) activities. The relationship between the chemical profile and bioactivities of RW should be established. However, due to the multi-compound system of TCM, the material basis and molecular mechanisms involved in RW remain unclear. Therefore, it is important to develop modern and technical means to analyze the mechanism of RW in treating various diseases.
Network pharmacology is a new research method that integrates pharmacodynamics, pharmacokinetics, and network analysis. In recent years, it has been applied to elucidate the possible mechanism of TCM prescriptions in the treatment of various diseases from the perspective of proteomics systems (25-27). In particular, it has been used to characterize the interaction relationship of TCM in multi-components and multitargets, as well as to study the mechanism of multitarget compounds affecting the biological network of TCM (28,29). In this study, we explore the hub active ingredients and potential mechanisms of RW in AP based on network pharmacology and molecular docking. We present this article in accordance with the STREGA reporting checklist (available at https://jtd.amegroups.com/article/view/10.21037/jtd-23-1891/rc).
Methods
Schematic diagram
In this study, network pharmacology strategies, including drug similarity assessment, oral bioavailability prediction, multiple drug target prediction, and other network pharmacology techniques, were used to study the potential role of RW against AP mechanisms. The gene target network of RW was analyzed through network pharmacology, starting with the identification of active substances and key target genes whose expression is altered in AP and whose protein products are predicted to interact with active compounds in RW. Figure 1 presents the schematic diagram of the study design and workflow. We investigated the mechanism of action of RW using network pharmacology and molecular docking methods.
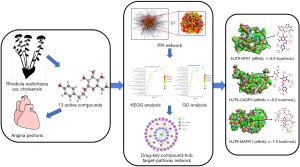
Chemical ingredient database building
A total of 83 RW compounds were collected through literature mining. Then, their biomolecular activities were viewed using the PubChem database (https://pubchem.ncbi.nlm.nih.gov) (30). Finally, the standard simplified molecular-input standard delay format (SDF) and the structural information of 42 compounds were obtained, including that of salidroside, tyrosol, and quercetin, among others (31-33).
Screening of active compounds of RW
The SDF format file was uploaded under the item “Chemical Ingredients Database Building” to the Swiss ADME platform (http://www.swissadme.ch) (34,35), a platform for predicting the relevant parameters of the absorption and drug-like properties of candidate compounds. First, we set the gastrointestinal (GI) absorption as “High” for the conditions under which the drug can be absorbed to screen for active compounds with good oral bioavailability; second, we set five types of drug predictability (Lipinski, Ghose, Veber, Egan, Muegge), and there were three or more compounds that appeared with “Yes” in the results, which could be considered the active compounds.
Active compound target prediction
The SDF format file was imported into the Swiss Target Prediction (http://www.Swisstargetprediction.ch/) (36,37) and PharmMapper (http://www.lilab-ecust.cn/pharmmapper/) (38-40) platforms with the property set to “Homo sapiens”, in order to collect all the predicted targets and removing duplication.
Prediction of known therapeutic targets
Genes associated with AP were collected and screened from the GeneCards database (http://www.genecards.org/) (41) and DigSee database (http://210.107.182.61/geneSearch/) (42). We searched GeneCards and DigSee for data using the keywords “angina pectoris” with the species limited to “Homo sapiens”. Then, the top 1,000 target genes were selected from the GeneCards database, and 541 targets were obtained from the DigSee database. Finally, 1,297 genes were collected after removal of duplicates. To obtain the potential target genes of RW that played a major role in AP, the AP-related genes were compared with potential gene targets of the active components.
Network construction
A network analysis was performed to scientifically determine the complex relationship between AP-related compounds and targets. Subsequently, based on the protein-protein interactions (PPIs), we linked the putative targets of RW, the AP-related targets, and interactional proteins together. Then, to illustrate the relationship between the possible targets of RW and known targets of AP, a drug-compound-target-disease network was constructed and visualized using Cytoscape software (version 3.7.2) (26). The PPI network of the interaction between RW and AP was obtained via Search Tool for the Retrieval of Interacting Genes/Proteins (STRING) software (http://string-db.org/cgi/input.pl), in which the limiting conditions were “Homo sapiens” and a confidence score ≥0.4. The subsequent results were saved in tab separated values (TSV) format and imported into Cytoscape software to visualize and analyze the interaction network. We used the style function from the statistics tool in Cytoscape to set the node size and color settings to reflect the size of the degree and the thickness of the edge of the comprehensive score to obtain the final protein interaction network. Degree refers to the number of links to node I and is usually used to describe the topological importance of proteins in the network. Therefore, we used cytoHubba to select the central gene with the degree value as the tangent point to analyze the pharmacological effects of key targets.
Gene Ontology (GO) and Kyoto Encyclopedia of Genes and Genomes (KEGG) pathway enrichment
GO and KEGG pathway enrichment analyses were executed on the candidate targets using Database for Annotation, Visualization and Integrated Discovery (DAVID) 6.8 (43). GO gene enrichment analysis included 3 categories: biological process (BP), molecular function (MF), and cell component (CC). With P≤0.05 as the truncated value, the results were calculated using bilateral hypergeometric tests, including the identification of the enriched GO terms and the localization of the biological and MFs of these proteins. Finally, the bubble chart was plotted using the ImageGP platform (http://www.ehbio.com/ImageGP/index.php/Home/Index/index.html) (44).
Molecular docking simulation
Core targets were obtained from the PPI network for molecular docking. Initially, AutoDockTools 1.5.6 was employed to set the number of rotatable bonds for the 12 small molecule compounds. Subsequently, protein conformation was determined in the Protein Data Bank (PDB; https://www.rcsb.org/) (45-47) database. The screening conditions were set as follows: (I) the protein structure was obtained by X-crystal diffraction; (II) the crystal resolution of the protein was less than 2.5 Å; (III) the species was Homo sapiens; and (IV) association action models were constructed with the STRING and PDB databases. Based on the above conditions, a total of 11 core target protein PDB IDs were gathered. Additionally, PyMOL 2.7 (https://pymol.org/2/) and AutoDockTools were applied to not only remove water molecules and proligand small molecules but also to hydrogenate and charge them. Finally, molecular docking calculations were performed using AutoDock Vina 1.1.2, and PyMOL and LigPlot+ software was used to visualize the docking results.
Results
Target screening of RW and AP
A total of 83 chemical ingredients were obtained from the RW according to related literature studies. After removal of duplicates, 26 chemical ingredients (Table 1) and 671 corresponding targets of RW were acquired, and 1,297 therapeutic targets for AP were obtained from the GeneCards database and DigSee database (Figure 2A). Then, the ultimate gene targets of RW acting on AP were obtained by mapping these targets to the components of the disease targets. As shown in Figure 2B, 218 target genes corresponding to RW candidate compounds of AP were obtained for further research.
Table 1
No. | Mol ID | Compound | Formula | Chemical structure |
---|---|---|---|---|
1 | HJT1 | Quercetin | C15H10O7 | ![]() |
2 | HJT2 | Herbacetin | C15H10O7 | ![]() |
3 | HJT3 | Cyanidin | C15H11O6+ | ![]() |
4 | HJT4 | Kaempferol | C15H10O6 | ![]() |
5 | HJT5 | Anthocyanin | C15H11O+ | ![]() |
6 | HJT6 | Cinnamic alcohol | C9H10O | ![]() |
7 | HJT7 | Rosin | C15H20O6 | ![]() |
8 | HJT8 | Salidroside | C14H20O7 | ![]() |
9 | HJT9 | Tyrosol | C8H10O2 | ![]() |
10 | HJT10 | p-Hydroxy-cinnamic acid | C9H8O3 | ![]() |
11 | HJT11 | Ferulic acid | C10H10O4 | ![]() |
12 | HJT12 | Rosiridol | C10H18O2 | ![]() |
13 | HJT13 | p-hydroxyphenethyl anisate | C16H16O4 | ![]() |
14 | HJT14 | 8-hydroxypinoresinol | C20H22O7 | ![]() |
15 | HJT15 | Methyl trans-cinnamate | C10H10O2 | ![]() |
16 | HJT16 | Paeonol | C9H10O3 | ![]() |
17 | HJT17 | Syringate | C9H10O5 | ![]() |
18 | HJT18 | Vanillin | C8H8O3 | ![]() |
19 | HJT19 | 2-furoic acid | C5H4O3 | ![]() |
20 | HJT20 | Tricin | C17H14O7 | ![]() |
21 | HJT21 | p-hydroxycinnamic acid | C9H8O3 | ![]() |
22 | HJT22 | β-D-phenyl glucopyranoside | C12H16O6 | ![]() |
23 | HJT23 | Picein | C14H18O7 | ![]() |
24 | HJT24 | Coniferin | C16H22O8 | ![]() |
25 | HJT25 | Scaphopetalone | C21H26O6 | ![]() |
26 | HJT26 | Berchemol | C20H24O7 | ![]() |
RW, Rhodiola wallichiana var. cholaensis; Mol, molecule.
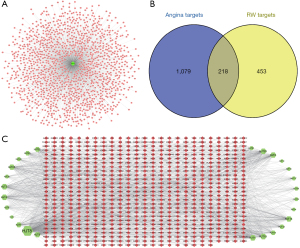
Network construction and result analysis
TCM exerts its therapeutic role mainly through the synergistic effect between compounds and targets. To illustrate the potential mechanism of this synergistic effect of RW on AP, it is necessary to understand the effects of each component in RW on its target proteins. Based on the network analysis of compounds and putative targets in Cytoscape 3.7.2, as shown in Figure 2C, our study showed that the network was composed of 1,754 edges and 697 nodes, among which 26 were component nodes and 671 were target nodes. It was clear that the target genes with high degree and mediated centrality were most important to the antianginal effect of RW in the network.
Construction and analysis of the PPI network
To further investigate the potential mechanism of action of RW in the treatment of AP, target genes acting on the corresponding components were submitted to the STRING database, a subsequent PPI network was constructed, and then highly reliable target protein interaction data sets with a score >0.7 were selected. A single protein is unlikely to perform a specific function, and proteins often interact with each other to form large molecular complexes that perform their biological functions. Thus, exploring and constructing a PPI network is key to understanding the BPs and biological functions of cells. The PPI network (Figure 3) file obtained was imported into Cytoscape software. After adjusting the parameters, we selected 70 key genes that were related to the various pathogenic processes of AP according to the degree.
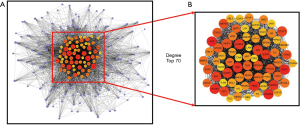
GO functional analysis
A total of 70 potential genes associated with AP were analyzed in the DAVID panel. We undertook GO enrichment analyses of 70 targets to explore their general functions. As shown in Figure 4, the GO analysis results showed the key predictive targets of RW acting on AP, and 20 GO items with low P values and more enrichment targets were enriched. Our results showed that these predictive targets were mainly involved in cell signal transduction, protein autophosphorylation, and positive regulation of cell proliferation, indicating that RW may regulate the biological function of AP through these pathways, thus playing a key role in treatment of AP.
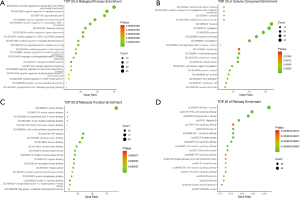
KEGG signaling pathway analysis
To identify the relevant signaling pathways involved in the antianginal effect of RW, DAVID analysis was performed. A total of 34 KEGG signaling pathways were obtained, and 27 pathways were associated with AP. To show the results of the signaling pathways intuitively and explicitly, a bubble diagram was drawn, as seen in Figure 4D; here, the bubble scale represents the number of genes, and the depth of the bubble color represents the P value; the change in color from green to red represents the P value from low to high, and the size of the nodes indicates how many target genes are associated.
The KEGG analysis results indicated multiple channels and mechanisms of action of RW against AP.
The top 20 pathways with lower P values and more gene enrichment are listed in Figure 4D and include the PI3K-Akt signaling pathway, HIF-1 signaling pathway, Ras signaling pathway, thyroid hormone signaling pathway, and Toll-like receptor signaling pathway. These signaling pathways are closely related to the anti-AP effects of RW. To elucidate their interactions, we established a graphical network containing the main chemical-target-signaling pathway of RW (Figure 5).
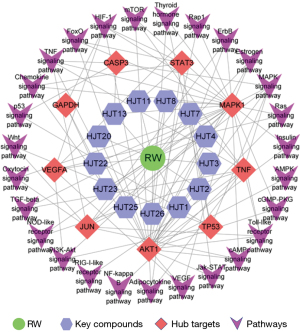
Molecular docking simulation
In this study, nine potential targets with 13 corresponding compounds were simulated with molecular docking, and the docking results were analyzed. The analysis revealed higher-affinity results for compound and hub targets (Table 2). Using PyMOL software, we found that 13 compounds could enter the active pocket of the protein. HJT8 (salidroside; Figure 6A) was used as an example for analysis. The salidroside small molecule forms four hydrogen bonds with Lys52, Asn152, Ser151, and Met106 residues and has a higher affinity (affinity =−7.5 kcal/mol) with MAPK1 (Figure 6B).
Table 2
Target protein | PDB ID | Active ingredient | Docked complex of protein and ligand | Affinity (kcal/mol) |
---|---|---|---|---|
AKT1 | 1UNQ | Quercetin | ![]() |
−6.4 |
Herbacetin | ![]() |
−6.2 | ||
Anthocyanidins | ![]() |
−5.6 | ||
Kaempferol | ![]() |
−6.2 | ||
Salidroside | ![]() |
−5.8 | ||
Tricin | ![]() |
−5.9 | ||
TNF | 5UUI | Rosin | ![]() |
−6.8 |
TP53 | 6IUA | Rosin | ![]() |
−6.8 |
MAPK1 | 2OJJ | Salidroside | ![]() |
−7.5 |
p-hydroxyphenethyl anisate | ![]() |
−6.9 | ||
Benzyl alcohol-O-β-D-pyran glycosidase | ![]() |
−5.9 | ||
l-picein | ![]() |
−6.4 | ||
Scaphopetalone | ![]() |
−7.5 | ||
Berchemol | ![]() |
−7.1 | ||
CASP3 | 1RHM | Salidroside | ![]() |
−6.0 |
p-hydroxyphenethyl anisate | ![]() |
−6.4 | ||
GAPDH | 6YND | Benzyl alcohol-O-β-D-pyran glycosidase | ![]() |
−7.3 |
l-picein | ![]() |
−7.8 | ||
c-JUN | 5T01 | Scaphopetalone | ![]() |
−5.8 |
VEGFA | 4ZFF | Benzyl alcohol-O-β-D-pyran glycosidase | ![]() |
−6.7 |
STAT3 | 1BJ1 | Ferulic acid | ![]() |
−5.8 |
PDB, Protein Data Bank.
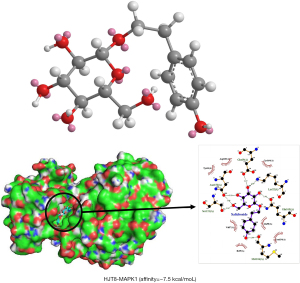
Discussion
TCM, a complex, mixed system of multiple ingredients and multiple targets, has traditionally been used to prevent and treat various cardiovascular diseases (CVDs) (33,48,49). RW has a high clinical value as a traditional Chinese medicine in relieving AP. Chu et al. (50) included seven randomized controlled trials in 662 patients with stable angina and found that the combination of oral/intravenous infusion of RW provided good relief of angina compared with conventional Western treatment. Man et al. (5) included 18 randomized controlled trials involving 1,679 patients and found that RW adjuvant therapy significantly reduced the frequency of angina attacks by ≥80%, the weekly frequency of angina attacks, and significantly improved abnormal electrocardiograms. In addition, it significantly reduced whole blood viscosity, plasma viscosity, and serum levels of fibrinogen. Although RW can effectively relieve AP, its pharmacological mechanism of action remains unclear. Consequently, in the present study, a pharmacology network method was used to identify bioactive compounds, potential targets, and the pathways modulated by these compounds in the RW treatment of AP.
Nine potential hub targets were identified based on selection and network topology analysis, including VEGFA, GAPDH, TP53, AKT1, CASP3, STAT3, TNF, MAPK1 and JUN. Numerous studies have shown that the targets mentioned above are mainly involved in the protection of the vascular endothelium, as well as the regulation of glucose metabolism, cellular processes, inflammatory responses, and cellular signal transduction. Vascular endothelial growth factor (VEGF), a strong pro-angiogenesis cytokine, is secreted by vascular endothelial cells, which could increase the permeability of microvessels and venules and promote angiogenesis, thus improving myocardial hypoxia and relieving AP (51-53). A recent study has demonstrated that the inflammatory response is related to the occurrence of CVDs such as coronary heart disease, which may cause local endothelial activation, atherosclerotic plaque rupture, and then thrombosis formation or rupture, leading to AP and myocardial infarction (54). Tumor necrosis factor-alpha (TNF-α) usually appears in the early stage of inflammatory reaction, and plays an important role in cell function regulation, immunity and inflammatory reaction. It can regulate atherosclerotic plaque and coronary heart disease by affecting vascular endothelial function and vascular remodeling (55). The study has shown that proinflammatory cytokines are significantly increased in patients with AP compared to healthy individuals. Meanwhile, it is important to note that this increased inflammatory activity may be related to the pathogenesis of AP. 1-Deoxynojirimycin (DNJ) significantly improves angina attack frequency by reducing the levels of inflammatory cytokines, including TNF-α (56). AKT1 is an important protein in the PI3K pathway that can regulate cell apoptosis, proliferation, and antioxidant activity (57,58). Han et al. (59) demonstrated that hypericin can reduce the inflammatory response by activating phosphorylated protein kinase B (PKB) and reducing TNF-α and interleukin (IL)-6 activity, thus alleviating myocardial ischemia-reperfusion injury. The p53 gene is an important apoptosis-related gene can be divided into two types: wild type (wp53) and mutant type (mp53). The mutant p53 gene can promote cell growth and participate in the occurrence of various tumors. The main function of the wild-type p53 gene is to participate in the negative regulation of cell growth, limiting cell growth and division. In recent years, studies have found that the p53 gene is not only associated with the occurrence and development of many tumors but also participates in the occurrence of apoptosis in the cardiovascular system (60,61). JUN is the heterodimer form of activating protein 1 (AP-1). Previous study has demonstrated that c-JUN can induce the production of adhesion factors in endothelial cells and increase the expression of chemokines and the formation of foam cells, thus promoting the formation and development of atherosclerosis (62). MAPK1 belongs to the Ser-Thr kinase protein family and has been previously reported in different features of cardiac modelling and regulation of inflammation, cell proliferation, and differentiation (63,64). STAT3 is a latent transcription factor that was initially identified as a cytokine signaling transductor and is involved in a variety of BPs, such as cell proliferation (65), differentiation (66), and survival (67).
To understand the potential biological mechanism of RW against AP, GO and KEGG functional enrichment analyses of DAVID and KEGG were applied. Through KEGG pathway analysis (P<0.05), we identified 21 AP-related signaling pathways, including HIF-1, PI3K-Akt, MAPK, FoXO, TNF, Ras, and Toll-like receptor signaling pathways. Accordingly, these pathways may be involved in the progression of AP. Based on the P value, we chose the HIF-1 signaling pathway as the most likely candidate for further study. Hypoxia-inducible factor (HIF) is a transcriptional complex that responds to changes in oxygen and provides a master regulator for cells to coordinate changes in gene transcription. HIF acts on all mammalian cell types and is ancient in evolutionary terms. At the molecular level, the HIF complex contains an α subunit and a β subunit, both of which can be selected from several options. HIF-β subunits are composed and participate in heterogeneous reactions. The α subunit is regulated and unique to hypoxic reactions. Under hypoxic conditions, HIF-1α is induced and highly expressed, transferring from the cytoplasm to the nucleus and initiating downstream gene expression, such as that of erythropoietin and VEGF. HIF-1α can increase myocardial glucose intake and transportation to continuously provide the compensatory energy supply by regulating myocardial GLUT4 and PKM2 gene expression (68). HIF-1α also facilitates the activation of PDK1 and PDK4 as well as UCP2 to enhance mitochondrial oxidative phosphorylation (69). Moreover, NRF1 and TFAM play distinct roles in mitochondrial biogenesis (70), and the upregulation of NRF1 and TFAM promotes mitochondrial DNA synthesis in infarcted cardiac muscle (71). Therefore, the HIF-1 signaling pathway is activated in cardiomyocytes to produce continuous adenosine triphosphate (ATP) in adaptation to hypoxia by shifting myocardial metabolism substrate to glucose intake and transportation (72).
In this study, GO enrichment analysis was adopted. The targets were connected with the regulation of protein phosphorylation, nitric oxide biosynthesis, cell membrane region, platelet alpha granule, protein kinase, and protein phosphatase. Therefore, the results suggest that RW treats AP by participating in BP, CC, and MF.
Molecular docking analysis simulation provided a visual interpretation of the interaction between key compounds and their potential protein targets. For example, salidroside mainly forms 4 hydrogen bonds with Lys52, Asn152, Ser151, and Met106 A residues on MAPK1. Salidroside exerts various pharmacological effects, such as antifatigue, antioxidation, immune regulation, and free radical scavenging activities. In recent years, in vivo and in vitro experiments have proven that the compound has positive anticancer, anti-inflammatory, antioxidative, neuroprotective, myocardial-protective, liver-protective, and kidney-protective effects (73,74). In addition, it has been reported that salidroside can inhibit the release of lactate dehydrogenase (LDH), creatine-kinase (CK) and aspartate aminotransferase (AST) from human cardiomyocytes by increasing the expression of HIF-1α, increasing the content of superoxide dismutase (SOD), increasing the activity of human cardiomyocytes, and reducing the death and apoptosis of cells (75). Overall, it was speculated that the main composition of RW may play a significant role in the treatment of AP through hub targets in these top-ranking signaling pathways. However, some limitations of our study should be considered. For instance, the results are only based on the screening of already known chemical constituents of RW, related targets, and signaling pathways from the literature and existing databases. Consequently, more in-depth research is required to characterize the underlying mechanisms of RW in the treatment of angina.
At present, network pharmacology is mostly used in the screening of drug active ingredients, prediction of the mechanism of action of specific drugs, analysis of targets of main active ingredients, and development of combination drugs. As a research idea, network pharmacology can also be used to explain the compatibility rules of traditional Chinese medicine compounds and discover new indications of traditional Chinese medicine. Network pharmacology is becoming a powerful and attractive tool to reflect the multi-component and multi-target characteristics of traditional Chinese medicine. Based on network pharmacology methods, it can help explain many difficult problems in the material basis of traditional Chinese medicine efficacy.
Conclusions
Via network pharmacology and molecular docking virtual computing, 26 ingredients of RW and putative known therapeutic targets were collected, and the underlying mechanism of RW in the treatment of AP was explored. RW exerted treatment effects on AP by regulating nine hub targets: VEGFA, GAPDH, TP53, AKT1, CASP3, STAT3, TNF, MAPK1 and JUN. Based on the results of GO and KEGG pathway enrichment analysis, we found that these hub targets ameliorated AP by participating in protecting the vascular endothelium, regulating glucose metabolism, regulating cellular processes, regulating the inflammatory response, and participating in cell signal transduction, among other processes. In summary, this study used a network pharmacology approach to investigate the complex network relationships among multiple components, targets, and pathways of RW in the treatment of AP. The results of this study verify and predict the molecular mechanism of RW in AP at the system level, which may provide enlightenment for the mechanism of RW and other anti-AP Chinese medicines and promote the application of RW in the treatment of AP. However, our research results are based on computational analysis, and further experiments are needed to verify these hypotheses.
Acknowledgments
Funding: This study was supported by
Footnote
Reporting Checklist: The authors have completed the STREGA reporting checklist. Available at https://jtd.amegroups.com/article/view/10.21037/jtd-23-1891/rc
Peer Review File: Available at https://jtd.amegroups.com/article/view/10.21037/jtd-23-1891/prf
Conflicts of Interest: All authors have completed the ICMJE uniform disclosure form (available at https://jtd.amegroups.com/article/view/10.21037/jtd-23-1891/coif). The authors have no conflicts of interest to declare.
Ethical Statement: The authors are accountable for all aspects of the work in ensuring that questions related to the accuracy or integrity of any part of the work are appropriately investigated and resolved.
Open Access Statement: This is an Open Access article distributed in accordance with the Creative Commons Attribution-NonCommercial-NoDerivs 4.0 International License (CC BY-NC-ND 4.0), which permits the non-commercial replication and distribution of the article with the strict proviso that no changes or edits are made and the original work is properly cited (including links to both the formal publication through the relevant DOI and the license). See: https://creativecommons.org/licenses/by-nc-nd/4.0/.
References
- Kloner RA, Chaitman B. Angina and Its Management. J Cardiovasc Pharmacol Ther 2017;22:199-209. [Crossref] [PubMed]
- Abbasi M, Neishaboury M, Koohpayehzadeh J, et al. National Prevalence of Self-Reported Coronary Heart Disease and Chronic Stable Angina Pectoris: Factor Analysis of the Underlying Cardiometabolic Risk Factors in the SuRFNCD-2011. Glob Heart 2018;13:73-82.e1. [Crossref] [PubMed]
- Divakaran S, Loscalzo J. The Role of Nitroglycerin and Other Nitrogen Oxides in Cardiovascular Therapeutics. J Am Coll Cardiol 2017;70:2393-410. [Crossref] [PubMed]
- Yang Y, Li Y, Wang J, et al. Systematic Investigation of Ginkgo Biloba Leaves for Treating Cardio-cerebrovascular Diseases in an Animal Model. ACS Chem Biol 2017;12:1363-72. [Crossref] [PubMed]
- Man C, Dai Z, Fan Y. Dazhu Hongjingtian Preparation as Adjuvant Therapy for Unstable Angina Pectoris: A Meta-Analysis of Randomized Controlled Trials. Front Pharmacol 2020;11:213. [Crossref] [PubMed]
- Yu L, Qin Y, Wang Q, et al. The efficacy and safety of Chinese herbal medicine, Rhodiola formulation in treating ischemic heart disease: a systematic review and meta-analysis of randomized controlled trials. Complement Ther Med 2014;22:814-25. [Crossref] [PubMed]
- Chang PK, Yen IC, Tsai WC, et al. Protective Effects of Rhodiola Crenulata Extract on Hypoxia-Induced Endothelial Damage via Regulation of AMPK and ERK Pathways. Int J Mol Sci 2018;19:2286. [Crossref] [PubMed]
- Battistelli M, De Sanctis R, De Bellis R, et al. Rhodiola rosea as antioxidant in red blood cells: ultrastructural and hemolytic behaviour. Eur J Histochem 2005;49:243-54.
- Schriner SE, Avanesian A, Liu Y, et al. Protection of human cultured cells against oxidative stress by Rhodiola rosea without activation of antioxidant defenses. Free Radic Biol Med 2009;47:577-84. [Crossref] [PubMed]
- Skopńska-Rózewska E, Wójcik R, Siwicki AK, et al. The effect of Rhodiola quadrifida extracts on cellular immunity in mice and rats. Pol J Vet Sci 2008;11:105-11.
- Seo WG, Pae HO, Oh GS, et al. The aqueous extract of Rhodiola sachalinensis root enhances the expression of inducible nitric oxide synthase gene in RAW264.7 macrophages. J Ethnopharmacol 2001;76:119-23. [Crossref] [PubMed]
- Cheng YZ, Chen LJ, Lee WJ, et al. Increase of myocardial performance by Rhodiola-ethanol extract in diabetic rats. J Ethnopharmacol 2012;144:234-9. [Crossref] [PubMed]
- Wang J, Rong X, Li W, et al. Rhodiola crenulata root ameliorates derangements of glucose and lipid metabolism in a rat model of the metabolic syndrome and type 2 diabetes. J Ethnopharmacol 2012;142:782-8. [Crossref] [PubMed]
- Qu ZQ, Zhou Y, Zeng YS, et al. Protective effects of a Rhodiola crenulata extract and salidroside on hippocampal neurogenesis against streptozotocin-induced neural injury in the rat. PLoS One 2012;7:e29641. [Crossref] [PubMed]
- Palumbo DR, Occhiuto F, Spadaro F, et al. Rhodiola rosea extract protects human cortical neurons against glutamate and hydrogen peroxide-induced cell death through reduction in the accumulation of intracellular calcium. Phytother Res 2012;26:878-83. [Crossref] [PubMed]
- Gao L, Wu C, Liao Y, et al. Herba Rhodiolae alleviates depression via the BDNF/TrkB-GSK-3β signaling pathway. Ann Transl Med 2021;9:1758. [Crossref] [PubMed]
- Chen QG, Zeng YS, Qu ZQ, et al. The effects of Rhodiola rosea extract on 5-HT level, cell proliferation and quantity of neurons at cerebral hippocampus of depressive rats. Phytomedicine 2009;16:830-8. [Crossref] [PubMed]
- Panossian A, Wikman G, Kaur P, et al. Adaptogens exert a stress-protective effect by modulation of expression of molecular chaperones. Phytomedicine 2009;16:617-22. [Crossref] [PubMed]
- Lee SY, Li MH, Shi LS, et al. Rhodiola crenulata Extract Alleviates Hypoxic Pulmonary Edema in Rats. Evid Based Complement Alternat Med 2013;2013:718739. [Crossref] [PubMed]
- Lee SY, Shi LS, Chu H, et al. Rhodiola crenulata and Its Bioactive Components, Salidroside and Tyrosol, Reverse the Hypoxia-Induced Reduction of Plasma-Membrane-Associated Na,K-ATPase Expression via Inhibition of ROS-AMPK-PKC ξ Pathway. Evid Based Complement Alternat Med 2013;2013:284150. [Crossref] [PubMed]
- Bayliak MM, Lushchak VI. The golden root, Rhodiola rosea, prolongs lifespan but decreases oxidative stress resistance in yeast Saccharomyces cerevisiae. Phytomedicine 2011;18:1262-8. [Crossref] [PubMed]
- Lee FT, Kuo TY, Liou SY, et al. Chronic Rhodiola rosea extract supplementation enforces exhaustive swimming tolerance. Am J Chin Med 2009;37:557-72. [Crossref] [PubMed]
- Liu Z, Li X, Simoneau AR, et al. Rhodiola rosea extracts and salidroside decrease the growth of bladder cancer cell lines via inhibition of the mTOR pathway and induction of autophagy. Mol Carcinog 2012;51:257-67. [Crossref] [PubMed]
- Shih CD, Kuo DH, Huang CW, et al. Autonomic nervous system mediates the cardiovascular effects of Rhodiola sacra radix in rats. J Ethnopharmacol 2008;119:284-90. [Crossref] [PubMed]
- Xu T, Li S, Sun Y, et al. Systematically characterize the absorbed effective substances of Wutou Decoction and their metabolic pathways in rat plasma using UHPLC-Q-TOF-MS combined with a target network pharmacological analysis. J Pharm Biomed Anal 2017;141:95-107. [Crossref] [PubMed]
- Zhang GX, Zhang YY, Zhang XX, et al. Different network pharmacology mechanisms of Danshen-based Fangjis in the treatment of stable angina. Acta Pharmacol Sin 2018;39:952-60. [Crossref] [PubMed]
- Guo S, Wu J, Zhou W, et al. Investigating the multi-target pharmacological mechanism of danhong injection acting on unstable angina by combined network pharmacology and molecular docking. BMC Complement Med Ther 2020;20:66. [Crossref] [PubMed]
- Wang X, Wang ZY, Zheng JH, et al. TCM network pharmacology: A new trend towards combining computational, experimental and clinical approaches. Chin J Nat Med 2021;19:1-11. [Crossref] [PubMed]
- Chen YY, Nan JY, Li HX, et al. Deciphering potential pharmacological mechanisms of Danhong injection to treat chronic stable angina based on drug response-related modules and genes. J Ethnopharmacol 2022;291:115125. [Crossref] [PubMed]
- Kim S, Thiessen PA, Bolton EE, et al. PubChem Substance and Compound databases. Nucleic Acids Res 2016;44:D1202-13. [Crossref] [PubMed]
- Wang XJ, Xie X, Luo X, et al. Chemical constituents from Rhodiola wallichiana var. cholaensis (I). Chin Tradit Herb Drugs 2015;46:3471-4.
- Liu Y, Chen C, Qiu J, et al. Characterization of the chemical constituents in Hongjingtian injection by liquid chromatography quadrupole time-of-flight mass spectrometry. Biomed Chromatogr 2019;33:e4446. [Crossref] [PubMed]
- Hao P, Jiang F, Cheng J, et al. Traditional Chinese Medicine for Cardiovascular Disease: Evidence and Potential Mechanisms. J Am Coll Cardiol 2017;69:2952-66. [Crossref] [PubMed]
- Daina A, Michielin O, Zoete V. SwissADME: a free web tool to evaluate pharmacokinetics, drug-likeness and medicinal chemistry friendliness of small molecules. Sci Rep 2017;7:42717. [Crossref] [PubMed]
- Daina A, Michielin O, Zoete V. iLOGP: a simple, robust, and efficient description of n-octanol/water partition coefficient for drug design using the GB/SA approach. J Chem Inf Model 2014;54:3284-301. [Crossref] [PubMed]
- Daina A, Michielin O, Zoete V. SwissTargetPrediction: updated data and new features for efficient prediction of protein targets of small molecules. Nucleic Acids Res 2019;47:W357. [Crossref] [PubMed]
- Gfeller D, Michielin O, Zoete V. Shaping the interaction landscape of bioactive molecules. Bioinformatics 2013;29:3073-9. [Crossref] [PubMed]
- Liu X, Ouyang S, Yu B, et al. PharmMapper server: a web server for potential drug target identification using pharmacophore mapping approach. Nucleic Acids Res 2010;38:W609. [Crossref] [PubMed]
- Wang X, Shen Y, Wang S, et al. PharmMapper 2017 update: a web server for potential drug target identification with a comprehensive target pharmacophore database. Nucleic Acids Res 2017;45:W356. [Crossref] [PubMed]
- Wang X, Pan C, Gong J, et al. Enhancing the Enrichment of Pharmacophore-Based Target Prediction for the Polypharmacological Profiles of Drugs. J Chem Inf Model 2016;56:1175-83. [Crossref] [PubMed]
- Stelzer G, Rosen N, Plaschkes I, et al. The GeneCards Suite: From Gene Data Mining to Disease Genome Sequence Analyses. Curr Protoc Bioinformatics 2016;54:1.30.1-1.30.33.
- Piñero J, Ramírez-Anguita JM, Saüch-Pitarch J, et al. The DisGeNET knowledge platform for disease genomics: 2019 update. Nucleic Acids Res 2020;48:D845-55. [Crossref] [PubMed]
- Sherman BT, Hao M, Qiu J, et al. DAVID: a web server for functional enrichment analysis and functional annotation of gene lists (2021 update). Nucleic Acids Res 2022;50:W216. [Crossref] [PubMed]
- Chen T, Liu YX, Huang L, Image GP. An easy-to-use data visualization web server for scientific researchers. iMeta 2022;1:e5.
- Burley SK, Bhikadiya C, Bi C, et al. RCSB Protein Data Bank: powerful new tools for exploring 3D structures of biological macromolecules for basic and applied research and education in fundamental biology, biomedicine, biotechnology, bioengineering and energy sciences. Nucleic Acids Res 2021;49:D437-51. [Crossref] [PubMed]
- Berman HM, Westbrook J, Feng Z, et al. The Protein Data Bank. Nucleic Acids Res 2000;28:235-42. [Crossref] [PubMed]
- Berman H, Henrick K, Nakamura H. Announcing the worldwide Protein Data Bank. Nat Struct Biol 2003;10:980. [Crossref] [PubMed]
- Hao DC, Xiao PG. Impact of Drug Metabolism/Pharmacokinetics and their Relevance Upon Traditional Medicine-based Cardiovascular Drug Research. Curr Drug Metab 2019;20:556-74. [Crossref] [PubMed]
- Shu Z, Wu T, Shahen M, et al. System-Pharmacology Dissection of Traditional Chinese herbs SINI Decoction for Treatment of Cardiovascular Diseases. An Acad Bras Cienc 2019;91:e20180424. [Crossref] [PubMed]
- Chu JF, Wu GW, Zheng GH, et al. A system review of randomized controlled trials on treating chronic stable angina by rhodiola. Zhongguo Zhong Xi Yi Jie He Za Zhi 2014;34:940-6.
- Liu G, Wang L, Lai L, et al. Peripheral serum VEGF level and its relavant factors in unstable angina pectoris patients. Chinese Journal of Geriatric Heart Brain and Vessel Diseases 2019;9:939-41.
- Li J, Cao GY, Zhang XF, et al. Chinese Medicine She-Xiang-Xin-Tong-Ning, Containing Moschus, Corydalis and Ginseng, Protects from Myocardial Ischemia Injury via Angiogenesis. Am J Chin Med 2020;48:107-26. [Crossref] [PubMed]
- Zhai S, Zhang XF, Lu F, et al. Chinese medicine GeGen-DanShen extract protects from myocardial ischemic injury through promoting angiogenesis via up-regulation of VEGF/VEGFR2 signaling pathway. J Ethnopharmacol 2021;267:113475. [Crossref] [PubMed]
- Li X, Guo D, Zhou H, et al. Pro-inflammatory Mediators and Oxidative Stress: Therapeutic Markers for Recurrent Angina Pectoris after Coronary Artery Stenting in Elderly Patients. Curr Vasc Pharmacol 2021;19:643-54. [Crossref] [PubMed]
- Bansal SS, Ismahil MA, Goel M, et al. Dysfunctional and Proinflammatory Regulatory T-Lymphocytes Are Essential for Adverse Cardiac Remodeling in Ischemic Cardiomyopathy. Circulation 2019;139:206-21. [Crossref] [PubMed]
- Ma Y, Lv W, Gu Y, et al. 1-Deoxynojirimycin in Mulberry (Morus indica L.) Leaves Ameliorates Stable Angina Pectoris in Patients With Coronary Heart Disease by Improving Antioxidant and Anti-inflammatory Capacities. Front Pharmacol 2019;10:569. [Crossref] [PubMed]
- Wang PW, Gao S, Xu YL, et al. Research progress on intervention of traditional Chinese medicine on coronary heart disease through mTOR signaling pathway. Chinese Traditional and Herbal Drugs 2018;49:5191-6.
- Chen B, Chen X, Liu C, et al. Co-expression of Akt1 and Wnt11 promotes the proliferation and cardiac differentiation of mesenchymal stem cells and attenuates hypoxia/reoxygenation-induced cardiomyocyte apoptosis. Biomed Pharmacother 2018;108:508-14. [Crossref] [PubMed]
- Han J, Xuan JL, Hu HR, et al. Protective effect against myocardial ischemia reperfusion injuries induced by hyperoside preconditioning and its relationship with PI3K/Akt signaling pathway in rats. Zhongguo Zhong Yao Za Zhi 2015;40:118-23.
- Men H, Cai H, Cheng Q, et al. The regulatory roles of p53 in cardiovascular health and disease. Cell Mol Life Sci 2021;78:2001-18. [Crossref] [PubMed]
- Wang H, He F, Liang B, et al. p53-Dependent LincRNA-p21 Protects Against Proliferation and Anti-apoptosis of Vascular Smooth Muscle Cells in Atherosclerosis by Upregulating SIRT7 via MicroRNA-17-5p. J Cardiovasc Transl Res 2021;14:426-40. [Crossref] [PubMed]
- Ji R, Gu Y, Zhang J, et al. TRIM7 promotes proliferation and migration of vascular smooth muscle cells in atherosclerosis through activating c-Jun/AP-1. IUBMB Life 2020;72:247-58. [Crossref] [PubMed]
- Wang Y, Jia Q, Zhang Y, et al. Amygdalin Attenuates Atherosclerosis and Plays an Anti-Inflammatory Role in ApoE Knock-Out Mice and Bone Marrow-Derived Macrophages. Front Pharmacol 2020;11:590929. [Crossref] [PubMed]
- Ruan Y, Li H, Cao X, et al. Inhibition of the lncRNA DANCR attenuates cardiomyocyte injury induced by oxygen-glucose deprivation via the miR-19a-3p/MAPK1 axis. Acta Biochim Biophys Sin (Shanghai) 2021;53:1377-86. [Crossref] [PubMed]
- Kumar S, Wang G, Zheng N, et al. HIMF (Hypoxia-Induced Mitogenic Factor)-IL (Interleukin)-6 Signaling Mediates Cardiomyocyte-Fibroblast Crosstalk to Promote Cardiac Hypertrophy and Fibrosis. Hypertension 2019;73:1058-70. [Crossref] [PubMed]
- Rajasingh J, Bord E, Hamada H, et al. STAT3-dependent mouse embryonic stem cell differentiation into cardiomyocytes: analysis of molecular signaling and therapeutic efficacy of cardiomyocyte precommitted mES transplantation in a mouse model of myocardial infarction. Circ Res 2007;101:910-8. [Crossref] [PubMed]
- Morris NL, Michael DN, Crotty KM, et al. Alcohol-Induced Glycolytic Shift in Alveolar Macrophages Is Mediated by Hypoxia-Inducible Factor-1 Alpha. Front Immunol 2022;13:865492. [Crossref] [PubMed]
- Cunha-Oliveira T, Ferreira LL, Coelho AR, et al. Doxorubicin triggers bioenergetic failure and p53 activation in mouse stem cell-derived cardiomyocytes. Toxicol Appl Pharmacol 2018;348:1-13. [Crossref] [PubMed]
- Chen Z, Tao S, Li X, Yao Q. Resistin destroys mitochondrial biogenesis by inhibiting the PGC-1α/ NRF1/TFAM signaling pathway. Biochem Biophys Res Commun 2018;504:13-8. [Crossref] [PubMed]
- Sheeran FL, Pepe S. Mitochondrial Bioenergetics and Dysfunction in Failing Heart. Adv Exp Med Biol 2017;982:65-80. [Crossref] [PubMed]
- Karwi QG, Uddin GM, Ho KL, et al. Loss of Metabolic Flexibility in the Failing Heart. Front Cardiovasc Med 2018;5:68. [Crossref] [PubMed]
- Shang H, Wang S, Yao J, et al. Salidroside inhibits migration and invasion of poorly differentiated thyroid cancer cells. Thorac Cancer 2019;10:1469-78. [Crossref] [PubMed]
- Hu R, Wang MQ, Ni SH, et al. Salidroside ameliorates endothelial inflammation and oxidative stress by regulating the AMPK/NF-κB/NLRP3 signaling pathway in AGEs-induced HUVECs. Eur J Pharmacol 2020;867:172797. [Crossref] [PubMed]
- Wang Y, Su Y, Lai W, et al. Salidroside Restores an Anti-inflammatory Endothelial Phenotype by Selectively Inhibiting Endothelial Complement After Oxidative Stress. Inflammation 2020;43:310-25. [Crossref] [PubMed]
- Zhang JB, Guo JX, Cardiology DO. Protective effect of salidroside on human myocardial cells during hypoxia injury and possible mechanism. China Journal of Modern Medicine 2017;8:21-6.