Overexpression of farnesoid X receptor in small airways contributes to epithelial to mesenchymal transition and COX-2 expression in chronic obstructive pulmonary disease
Introduction
Chronic obstructive pulmonary disease (COPD) is characterised by progressive airflow limitation mainly due to abnormal airway remodelling and inflammation. The narrowing of small airways leads to increased peripheral airway resistance of patients with COPD (1). Although mechanisms of airway remodelling in COPD are not yet clearly elucidated, epithelial-mesenchymal transition (EMT) has been implicated in this process (2,3). During EMT, airway epithelial cells can acquire a mesenchymal phenotype and transform into fibroblasts/myofibroblasts, which produce excessive ECM proteins. Elevated mesenchymal biomarkers including α-smooth muscle actin (α-SMA), vimentin and collagen type I, as well as decreased epithelial biomarkers E-cadherin, ZO-1 and cytokeratin have been observed in small airway epithelium from COPD patients. Moreover, EMT features of the bronchial epithelium are associated with the severity of airway limitation (3,4), suggesting the involvement of EMT in progression of COPD. The induction of EMT in human bronchial epithelial (HBE) cells was also observed upon the stimulation with cigarette smoke or TGF-β1 in vitro (3,5). Taken together, these findings indicate the involvement of EMT in airway remodelling of COPD. However, the underlying mechanisms of EMT in small airways (<2.0 mm in diameter) of COPD lungs remain to be further elucidated.
Cyclooxygenase-2 (COX-2), a key enzyme in the conversion of arachidonic acid into prostaglandins E2 (PGE2), is an important player in airway inflammation of COPD. The levels of COX-2 and PGE2 are significantly increased in COPD lungs, and the expression of PGE2 is associated with the severity of airflow limitation (6-8). Several types of lung cells, including airway epithelial cells and lung fibroblasts, are capable of producing COX-2 in response to toxic irritators such as cigarette smoke, bile acid aspiration and bacterial infection (9-11). Moreover, inhibition of COX-2 or COX-2-induced PGE2 was shown to attenuate airway inflammation and the development of emphysema in cigarette smoking (CS)-exposed rats (12,13), supporting a role for COX-2 in the pathobiology of COPD.
Farnesoid X receptor (FXR), a member of the nuclear receptor superfamily, is highly expressed in the liver and gastrointestinal tract where it regulates bile acid homeostasis, lipid and glucose metabolism (14,15). Unconjugated bile acids, such as chenodeoxycholic acid (CDCA), are the natural ligands that activate FXR. In addition, synthetic FXR agonists such as GW4064 have also been identified (16). Increasing evidences suggest that FXR is also expressed in non-classical bile acids target tissues with novel functions (17-19). For example, expression of FXR in the vasculature regulates the transport of cholesterol and vascular tension (15,20). FXR has been detected in human airway epithelial cell lines and pulmonary vascular endothelial cells (21,22), and it has been shown to protect lipopolysaccharide-induced acute lung inflammation and improve lung regeneration in animal model (23). Recently, we reported the increased FXR expression in idiopathic pulmonary fibrosis (IPF) lungs and its contribution to bile acids-induced alveolar EMT and lung fibroblast activation (24). These findings indicate that FXR may be involved in the pathophysiology of lung diseases. However, to date, the expression and potential role of FXR in COPD remains unclear.
This study aimed to examine FXR expression in small airway epithelium of COPD lungs and investigate whether FXR regulates EMT and COX-2 expression in HBE cells. Furthermore, factors regulating FXR expression in bronchial epithelial cells were also analyzed.
Methods
Patients
The human lung tissues in this study were obtained from patients undergoing lung cancer resection for a solitary peripheral carcinoma at Ren Ji Hospital, School of Medicine, Shanghai Jiao Tong University and Affiliated Hospital of Xuzhou Medical College between July 2010 and August 2015. The subjects were divided into three groups: non-smokers with normal lung function (NS), smokers with normal lung function (S), and smokers with COPD. Smokers were defined as those who have a smoking history of at least 10 pack years and COPD patients were diagnosed according to the Global Initiative for Chronic Obstructive Lung Disease (GOLD) guidelines. Patients were excluded if they met the following criteria: other systemic diseases; glucocorticoid treatment for 2 months before surgery; respiratory tract infections during the previous 6 weeks; received chemotherapy or radiotherapy. This study was approved by the local ethics committee. All subjects provided an informed consent.
Cell culture and reagents
HBE cells were obtained from American Type Culture Collection and cultured in DMEM with 10% fetal bovine serum (FBS; Gibco) in a humidified atmosphere containing 5% CO2 at 37 °C. Cells were serum-starved overnight in DMEM without FBS before various treatments.
Recombinant human TGF-β1, IL-4 and IL-13 was purchased from R&D Systems (Minneapolis, MN, USA). CDCA was purchased from Sigma (St. Louis, MO, USA), GW4064 and Z-guggulsterone were purchased from Santa Cruz Biotechnology (Santa Cruz, CA, USA).
Histopathology and immunohistochemistry
Histopathology of paraffin-embedded lung tissue sections were conducted using haematoxylin and eosin (H&E) staining. FXR expression in lung tissue was using immunohistochemical staining as previously described (25). The primary antibodies used for immunostaining were as follows: rabbit polyclonal anti-FXR antibody (1:100) and mouse monoclonal vimentin antibody (1:100) from Abcam, mouse monoclonal E-Cadherin antibody (1:100) and rabbit monoclonal COX-2 antibody (1:100) from BD Biosciences. Immunostaining without primary antibodies was used as negative controls. Semi-quantification of the immunostaining was performed using a visual scoring method as previously described (26). Staining intensity was scored as follows: 0= no staining; 1= moderate staining; 2= intense staining; 3= very intense staining. The percentage of immunostaining-positive cells was categorized into four groups: 0=0%; 1=1–25%; 2=26–50%; 3=51–75%; 4=76–100%. Staining scores were generated by multiplication of the percentage of positive bronchial epithelial cells and staining intensity. Ten random microscopic fields at high magnification from each slide were analyzed. Each field was individually assessed and the expression score was determined by obtaining the mean score of all the fields. Two pathologists blinded to the clinical data reviewed the histology slides independently.
Real-time polymerase chain reaction (PCR) amplification
Total RNA was extracted using TRIzol (Life Technologies, NY, USA), and reverse transcription was performed according to standard methods. Real-time PCR was performed by a ViiA™ 7 Real-Time PCR System (Applied Biosystems, Foster City, CA, USA). GAPDH was used as the loading control. The fold change of the SHP was calculated according to the 2−ΔΔCt method. The primers were as follows: SHP, F-AGGCCTCCAAGCCGCCTCCCACATTGGGC and R-GCAGGCTGGTCGGAAACTTGAGGGT; GAPDH, F-ACAGTCAGCCGCATCTTCTT and R-GACAAGCTTCCCGTTCTCAG.
Western blotting
Western Blotting was performed using standard protocols. In brief, cells were harvested by using SDS lysed buffer. Equal amounts of proteins among different treatment groups were electrophoresed in 10% SDS-PAGE gels and transferred to a nitrocellulose membrane. Membranes were blocked in 3% BSA and incubated subsequently with rabbit polyclonal FXR antibody (1:500), mouse monoclonal antibodies for α-SMA (1:5,000), vimentin (1:4,000) and GAPDH (1:10,000) from Abcam, mouse monoclonal E-Cadherin antibody (1:5,000) from BD Bioscience, and mouse monoclonal SHP (1:500) from Santa Cruz overnight at 4 °C. HRP-conjugated monoclonal goat anti-rabbit or anti-mouse IgG antibodies were used as the secondary antibody. Bands were visualized with ECL reagents (Thermo Scientific, Waltham, MA, USA). Densitometric scans were quantified using NIH Image J and values were presented as relative intensities compared to GAPDH.
Rat model of COPD model
Sprague-Dawley (SD) rats weighing 180–200 g, purchased from by Department of Laboratory Animal Sciences, Ren Ji Hospital, Shanghai Jiao Tong University School of Medicine, were divided into control and smoke-exposed group. The procedures for establishing rat model of COPD were done as previously described (27,28). Briefly, SD rats were placed in 6-L Perspex chambers and exposed to cigarette smoke (CS) generated from 18 cigarettes per day or room air. Cigarettes used in this study were non-filtered and contained 12 mg tar oil and 1.25 mg nicotine per cigarette (Da Qian Men; Shanghai Tobacco Factory, Shanghai, China). At the end of 8 weeks, all rats were killed and their lung tissues were fixed in formalin and embedded in paraffin. All experiments were approved by the Institutional Animal Care and Use Committee.
Statistical analyses
Experimental data were presented as means ± standard error mean (SEM) and SPSS 18.0 was used for statistical analysis. The associations between variables were analyzed by Pearson’s correlation coefficient. The differences among groups were examined by using Student’s t-test or ANOVA. Significance was accepted as P<0.05.
Results
Patient demographic characteristics
Demographic characteristics and pulmonary function functional evaluation of the study subjects are shown in Table 1. All patient groups exhibited a similar age range and BMI values. Compared with subjects without COPD, patients with COPD had lower FEV1% predicted and FEV1/FVC values.
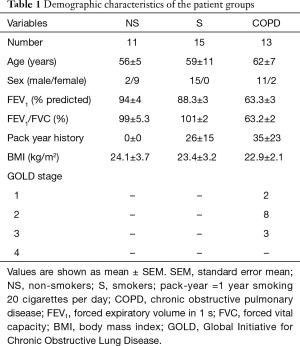
Full table
FXR expression is increased in small airways of human COPD lungs and correlated with lung function decline
Immunohistochemistry staining showed that FXR expression was significantly increased in the small airway epithelium from patients with COPD compared with non-smoker and smoker controls, and FXR was predominantly located to the nuclei of bronchial epithelial cells, but was also found in the cytoplasm. Moreover, a marked increase in FXR staining was found in the small airway epithelium of smoker controls compared with non-smokers (Figure 1A,B).
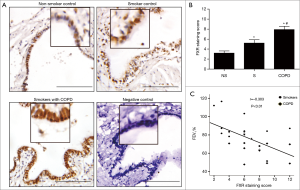
FEV1% of predicted is the most valuable parameter to assess the severity of airflow limitation in COPD patients. We next examined whether FXR expression in the small airways correlated with FEV1% of predicted. Correlation analysis performed in smokers without and with COPD showed that the staining scores of FXR in small airway epithelium were negatively related with FEV1% of predicted (r=−0.303, P<0.01, Figure 1C). These results indicate that overexpression of FXR in small airway epithelium may have a potential role in the progression of COPD.
FXR can be biologically activated in bronchial epithelial cells
To examine whether FXR is functional in airway epithelium, we evaluated the induction of expression of SHP, the known FXR target gene, in response to the primary bile acid CDCA (a natural FXR ligand) or GW4064 (a synthetic FXR agonist). Real-time PCR showed that both CDCA and GW4064 increased the levels of SHP mRNA in HBE cells. In contrast, FXR antagonist Z-Guggulsterone significantly inhibited CDCA-induced SHP mRNA expression in HBE cells (Figure 2A).
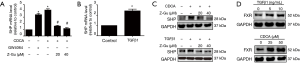
As FXR activation can also be regulated by some inflammatory mediators, we explored whether TGF-β1, the critical mediator in airway remodelling, could induce activation of FXR in HBE cells. We found that TGF-β1 also increased SHP mRNA expression in HBE cells (Figure 2B), suggesting the activation of FXR by TGF-β1 in HBE cells. The expression of SHP protein was also induced by CDCA or TGF-β1, which was dramatically inhibited by Z-Guggulsterone (Figure 2C). Furthermore, both CDCA and TGF-β1 increased FXR protein expression in HBE cells (Figure 2D). Together, these findings indicated that FXR can be biologically activated by CDCA and TGF-β1 in HBE cells.
Overexpression of FXR contributes to EMT changes in human small airway epithelium
Since EMT has been implicated in the process of airway remodelling in COPD, we next investigated whether FXR was involved in EMT of airway epithelium. We first assessed the expression of EMT biomarkers in small airway epithelium (<2.0 mm in diameter) from patients with lung resection. The immunohistochemistry staining revealed the downregulation of E-cadherin and upregulation of vimentin in small airway epithelium of COPD lungs compared with non-smoker and smoker controls, indicating the presence of active EMT (Figure 3A). Moreover, increased vimentin was also observed in small airway epithelium of smoker compared with non-smoker controls by scoring (Figure 3B).
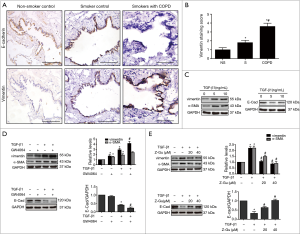
To investigate if FXR would regulate the airway EMT, HBE cells were stimulated with TGF-β1 (5 ng/mL) for 72 hours to induce EMT. As shown in Figure 3C, TGF-β1 significantly downregulated E-cadherin and upregulated α-SMA and vimentin in HBE cells, confirming the reliability of TGF-β1-induced EMT in HBE cells. Co-treatment of the FXR agonist GW4064 remarkably enhanced TGFβ1-induced changes in α-SMA, vimentin and E-cadherin in HBE cells, whereas it only slightly enhanced TGFβ1-induced α-SMA. In addition, GW4064 also slightly induced changes of EMT markers (Figure 3D). In contrast, co-treatment with FXR antagonist Z-Guggulsterone significantly reversed TGF-β1-induced changes in α-SMA, vimentin and E-cadherin in HBE cells (Figure 3E). These results indicate that FXR regulates EMT in human small airway epithelium.
Overexpression of FXR regulates the bile acid-induced COX-2 expression in human small airway epithelial cells
Gastroesophageal reflux disease (GERD) is recognized as a potential risk factor for exacerbation of COPD (29) and the bile acid aspiration has been reported to induce lung injury by promoting COX-2 expression in human alveolar epithelial cells (11). Therefore, we next explored whether FXR activation could regulate the bile acid-induced COX-2 expression in HBE cells. The immunohistochemistry showed enhanced COX-2 expression in small airway epithelium of COPD lungs compared to smoker or non-smoker controls, suggesting the overproduction of COX-2 in airways from patients with COPD. Furthermore, COX-2 staining scores were higher in smoker controls than that in non-smokers (Figure 4A,B). Western blotting analysis showed that stimulation of HBE cells with CDCA led to a significant increase in COX-2 expression in a dose and time-dependent manner (Figure 4C,D). When stimulated with GW4064, an enhanced COX-2 expression was also observed in HBE cells (Figure 4E). In contrast, pretreatment with FXR antagonist Z-Guggulsterone markedly abrogated CDCA-induced COX-2 expression (Figure 4F). Taken together, these results indicate that FXR contribute to the bile acid-induced COX-2 expression in HBE cells.
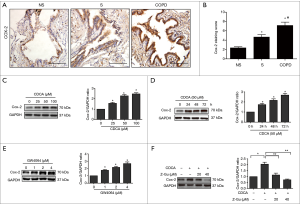
FXR expression is induced by inflammatory mediators in vitro and cigarette smoke exposure in vivo
Finally, we went on to investigate other factors regulating FXR expression in HBE cells. IL-4 and IL-13 are pleiotropic Th2 cytokines known to mediate airway remodelling and inflammation of COPD. Western blotting showed that stimulation with IL-4 or IL-13 induced FXR expression in cultured HBE cells (Figure 5A,B). To investigate the effect of CS on FXR expression in airways in vivo, we induced a rat COPD model by exposing rats to CS. HE staining showed more thickened bronchial wall and prominent inflammatory cell infiltration around airway and alveolar septa in CS-exposed rats (n=8) compared with controls (n=8). The immunohistochemical staining revealed the overexpression of FXR in small airway epithelium of lungs form CS-exposed rats compared to controls (Figure 5C,D). Together, these results indicate that FXR expression is induced by IL-4 and IL-13 in vitro and CS exposure in vivo.
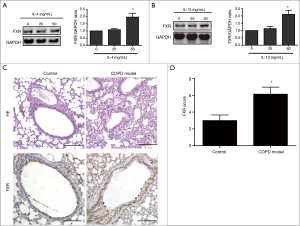
Discussion
In the present study, we demonstrated for the first time that FXR regulates EMT and COX-2 expression in the small airways of patients with COPD. We observed that FXR was increased in the small airway epithelium of patients with COPD compared with non-smokers and smokers with normal lung function. Furthermore, FXR expression in small airway epithelium was correlated with FEV1% predicted of smokers without COPD and smokers with COPD. Functional experiments indicate that FXR regulates TGF-β1-induced EMT and CDCA-induced COX-2 expression in HBE cells. In addition, FXR expression was induced by IL-4 and IL-13 in vitro and CS exposure in vivo. Taken together, these findings suggest that overexpression of FXR contributes to airway remodelling and inflammation in COPD.
FXR was reported to be distributed in a variety of tissues, with abundant levels in liver, gastrointestinal tract, and adrenal gland (14). A few in vitro studies demonstrated the expression of FXR in human airway epithelial cell lines and pulmonary endothelial cells, suggesting the potential role of FXR in lung diseases (21,22). Recently, we have demonstrated FXR expression in the alveolar epithelium, alveolar macrophages, and bronchial epithelial cells in normal lungs and upregulation of FXR in IPF lungs (24). However, up to date, the expression of FXR in COPD lungs has not been reported. In this study, we revealed that FXR expression was detected in human small airway epithelium and was upregulated in COPD lungs. Of note, overexpression of FXR in small airway was correlated with airflow limitation, indicating FXR may be involved in the development and progression of COPD.
EMT serves as an important source of the fibroblasts and myofibroblasts that arise during the course of airway fibrosis. The presence of EMT changes has been well-documented on larger airways in patients of COPD. However, airway remodelling in COPD, such as peribronchiolar fibrosis, is mainly located in small airways. Furthermore, the large and small airways have different anatomical and pathophysiological characteristics (30). Therefore, the role of active EMT in small airways remains to be elucidated. Our data demonstrated the downregulation of E-cadherin and upregulation of vimentin in small airway epithelium of the COPD lungs, indicating the active EMT in small airways of COPD patients. These results are consistent with previous findings that EMT contributed to remodelling of small airways in COPD (3,30,31). Up to date, there are limited data regarding the potential role of FXR in EMT. A recent study on pancreatic cancer demonstrated that overexpression of FXR promotes tumor migration and invasion, both of which are closely related to EMT (32). We have recently reported that FXR contributed to bile acid-induced EMT in alveolar epithelial cell line A549, suggesting the involvement of FXR in alveolar EMT (24). The present study demonstrated the contribution of FXR to EMT in small airways of COPD lungs as the following findings: Firstly, we found that TGF-β1, the strong inducer of EMT, could biologically activated FXR in HBE cells. Secondly, FXR agonist GW4064 could enhance TGF-β1-induced EMT in HBE cells, whereas FXR antagonist Z-Guggulsterone inhibited this process in HBE cells. To the best of our knowledge, this is the first study exploring the role of FXR in EMT in small airways. Since EMT features of airway are found to be associated with the severity of airway limitation (3,4), overexpression of FXR may contributed to airflow limitation in COPD patients through promoting EMT in small airways. Additional studies are needed to further clarify the underlying mechanisms.
Accumulating evidences suggest that airway epithelial cells can participate in persistent airway inflammation via the secretion of multiple inflammatory mediators, such as COX-2, in response to Cigarette smoke and other irritants. COX-2 is the main contributor to the production of pro-inflammatory prostanoids in airway inflammation and other inflammatory diseases (7,9,33). Similar to previous reports (6,7), our data also demonstrated the overexpression of COX-2 in small airways of COPD lungs. It has been reported that the bile acid CDCA promotes COX-2 expression in primary human alveolar epithelial cells (11), and FXR mediates bile acid-induced COX-2 expression in esophageal cancer cell lines (34). We further investigated whether bile acid also could induce COX-2 in airway epithelial cells and if FXR was involved in this process. We found that CDCA also promoted COX-2 expression in HBE cells, and this effect was greatly attributed to FXR activation since FXR agonist GW4064 also induced COX-2 expression and FXR antagonist Z-Guggulsterone markedly inhibited CDCA-induced COX-2 expression. It is well-known that GERD is common in patients with COPD and related to COPD exacerbations (29), however, the underlying mechanisms remain unclear. The gastric contents contain gastric acid and non-acid contents such as bile acids. Over a long time, gastric acid has been conceived as the dominant toxic agent in GER-associated lung diseases. However, the role of non-acid gastric contents is increasingly recognized in GERD-associated lung diseases (35-37). Our data indicated that bile acids aspiration may promote airway inflammation via FXR-mediated COX-2 expression, providing a novel insight of the association between GERD and COPD exacerbations. The optimal strategies for treating GERD in patients with COPD may involve targeting gastric acid and bile acids reflux, rather than acid suppression therapy alone. However, since bile acid aspiration was only found in a proportion of COPD patients, the contribution of FXR activation to airway inflammation in COPD would not be fully explained by bile acid aspiration. Additional studies are required to investigate whether other factors such as inflammatory mediators and environmental triggers, may be involved in FXR activation in the airways of COPD. In contrast to our findings, a recent study demonstrated that FXR protects against lipopolysaccharide-induced acute lung inflammation (23). Such contradictory effects of FXR may be related to various etiologies of this condition, and further investigations are needed.
In addition to overexpression in COPD lungs, FXR was also overexpressed in small airways from smokers compared with non-smokers. These findings indicated that both COPD and CS exposure itself might regulate FXR expression. Indeed, enhanced FXR in small airway epithelium form CS-exposed rats confirmed the induction of FXR by CS exposure in vivo. Furthermore, we demonstrated that the two inflammatory mediators IL-4 and IL-13 promoted FXR expression in HBE cells. The Th2 cytokines IL-4 and IL-13 are generally known to be the critical mediators in airways hyper-reactivity in asthma. However, both of these cytokines have been demonstrated to be involved in the development and progression of COPD (38). It has been reported that the expression of IL-4 and IL-13 was increased in airways of smokers with chronic bronchitis (39). In airways from patients with COPD, the upregulation of IL-4, IL-13 potentially stimulate the overexpression of FXR, which contributes to airway inflammation and remodelling of COPD. Therefore, there may exist a vicious cycle between FXR and persistent airway inflammation and remodelling in COPD. The hyperplasia of reprogrammed epithelial basal stem cell system in smoke exposed epithelium also play a role in COPD-relevant airway epithelial remodeling (40), which may affected FXR expression in small airway of COPD and CS-exposed rat lungs. However, our data indicated that upregulation of FXR was the “active player” in airway remodelling in COPD, rather than the subsequent response to airway remodelling. The underlying molecular mechanisms underlying the regulation of FXR expression remains to be further elucidated in additional studies.
There are some limitations of the current study. First, the strength of our findings in the present study is limited by the relatively small sample size for COPD, and further studies with larger sample sizes are needed. Despite this limitation, our data indicate the potential role of FXR in the development and progression of airway remodelling and inflammation in COPD. Second, the precise molecular mechanisms underlying the regulatory function of FXR on EMT and airway inflammation of COPD remain to be further elucidated.
In conclusion, overexpression of FXR in small airways of COPD lungs may contribute to airway remodelling and inflammation through regulating EMT and COX-2 expression. Both inflammatory mediators and cigarette smoke promote FXR expression in small airway epithelium. FXR would be a potential therapeutic target for future management of COPD.
Acknowledgements
We thank Drs. Meiyu Geng and Jing Ai (Shanghai Institute of Materia Medica, Chinese Academy of Sciences) and other members of Dr. Meiyu Geng’s laboratory for their assistance in lab work.
Funding: This study was supported in part by Grants from the National Natural Science Foundation of China (Grant No. 81274143).
Footnote
Conflicts of Interest: The authors have no conflicts of interest to declare.
Ethical Statement: This study was approved by the local ethics committee (approval number: 2013-042 and xyfy2010028). All subjects provided an informed consent.
References
- Hogg JC, Chu F, Utokaparch S, et al. The nature of small-airway obstruction in chronic obstructive pulmonary disease. N Engl J Med 2004;350:2645-53. [Crossref] [PubMed]
- Sohal SS, Walters EH. Role of epithelial mesenchymal transition (EMT) in chronic obstructive pulmonary disease (COPD). Respir Res 2013;14:120. [Crossref] [PubMed]
- Milara J, Peiró T, Serrano A, et al. Epithelial to mesenchymal transition is increased in patients with COPD and induced by cigarette smoke. Thorax 2013;68:410-20. [Crossref] [PubMed]
- Gohy ST, Hupin C, Fregimilicka C, et al. Imprinting of the COPD airway epithelium for dedifferentiation and mesenchymal transition. Eur Respir J 2015;45:1258-72. [Crossref] [PubMed]
- Zhang M, Zhang Z, Pan HY, et al. TGF-beta1 induces human bronchial epithelial cell-to-mesenchymal transition in vitro. Lung 2009;187:187-94. [Crossref] [PubMed]
- Xaubet A, Roca-Ferrer J, Pujols L, et al. Cyclooxygenase-2 is up-regulated in lung parenchyma of chronic obstructive pulmonary disease and down-regulated in idiopathic pulmonary fibrosis. Sarcoidosis Vasc Diffuse Lung Dis 2004;21:35-42. [PubMed]
- Chen Y, Chen P, Hanaoka M, et al. Enhanced levels of prostaglandin E2 and matrix metalloproteinase-2 correlate with the severity of airflow limitation in stable COPD. Respirology 2008;13:1014-21. [PubMed]
- Montuschi P, Kharitonov SA, Ciabattoni G, et al. Exhaled leukotrienes and prostaglandins in COPD. Thorax 2003;58:585-8. [Crossref] [PubMed]
- N'Guessan PD, Temmesfeld-Wollbrück B, Zahlten J, et al. Moraxella catarrhalis induces ERK- and NF-kappaB-dependent COX-2 and prostaglandin E2 in lung epithelium. Eur Respir J 2007;30:443-51. [Crossref] [PubMed]
- Anto RJ, Mukhopadhyay A, Shishodia S, et al. Cigarette smoke condensate activates nuclear transcription factor-kappaB through phosphorylation and degradation of IkappaB(alpha): correlation with induction of cyclooxygenase-2. Carcinogenesis 2002;23:1511-8. [Crossref] [PubMed]
- Su KC, Wu YC, Chen CS, et al. Bile acids increase alveolar epithelial permeability via mitogen-activated protein kinase, cytosolic phospholipase A2, cyclooxygenase-2, prostaglandin E2 and junctional proteins. Respirology 2013;18:848-56. [Crossref] [PubMed]
- Roh GS, Yi CO, Cho YJ, et al. Anti-inflammatory effects of celecoxib in rat lungs with smoke-induced emphysema. Am J Physiol Lung Cell Mol Physiol 2010;299:L184-91. [Crossref] [PubMed]
- Gu W, Song L, Li XM, et al. Mesenchymal stem cells alleviate airway inflammation and emphysema in COPD through down-regulation of cyclooxygenase-2 via p38 and ERK MAPK pathways. Sci Rep 2015;5:8733. [Crossref] [PubMed]
- Wang YD, Chen WD, Moore DD, et al. FXR: a metabolic regulator and cell protector. Cell Res 2008;18:1087-95. [Crossref] [PubMed]
- Bishop-Bailey D, Walsh DT, Warner TD. Expression and activation of the farnesoid X receptor in the vasculature. Proc Natl Acad Sci U S A 2004;101:3668-73. [Crossref] [PubMed]
- Porez G, Prawitt J, Gross B, et al. Bile acid receptors as targets for the treatment of dyslipidemia and cardiovascular disease. J Lipid Res 2012;53:1723-37. [Crossref] [PubMed]
- Mencarelli A, Renga B, Distrutti E, et al. Antiatherosclerotic effect of farnesoid X receptor. Am J Physiol Heart Circ Physiol 2009;296:H272-81. [Crossref] [PubMed]
- Khurana S, Raufman JP, Pallone TL. Bile acids regulate cardiovascular function. Clin Transl Sci 2011;4:210-8. [Crossref] [PubMed]
- Zhang X, Huang S, Gao M, et al. Farnesoid X receptor (FXR) gene deficiency impairs urine concentration in mice. Proc Natl Acad Sci U S A 2014;111:2277-82. [Crossref] [PubMed]
- Li YT, Swales KE, Thomas GJ, et al. Farnesoid x receptor ligands inhibit vascular smooth muscle cell inflammation and migration. Arterioscler Thromb Vasc Biol 2007;27:2606-11. [Crossref] [PubMed]
- Hendrick SM, Mroz MS, Greene CM, et al. Bile acids stimulate chloride secretion through CFTR and calcium-activated Cl- channels in Calu-3 airway epithelial cells. Am J Physiol Lung Cell Mol Physiol 2014;307:L407-18. [Crossref] [PubMed]
- He F, Li J, Mu Y, et al. Downregulation of endothelin-1 by farnesoid X receptor in vascular endothelial cells. Circ Res 2006;98:192-9. [Crossref] [PubMed]
- Zhang L, Li T, Yu D, et al. FXR protects lung from lipopolysaccharide-induced acute injury. Mol Endocrinol 2012;26:27-36. [Crossref] [PubMed]
- Chen B, Cai HR, Xue S, et al. Bile acids induce activation of alveolar epithelial cells and lung fibroblasts through farnesoid X receptor-dependent and independent pathways. Respirology 2016;21:1075-80. [Crossref] [PubMed]
- Kranenburg AR, de Boer WI, Alagappan VK, et al. Enhanced bronchial expression of vascular endothelial growth factor and receptors (Flk-1 and Flt-1) in patients with chronic obstructive pulmonary disease. Thorax 2005;60:106-13. [Crossref] [PubMed]
- Nagashio R, Ueda J, Ryuge S, et al. Diagnostic and prognostic significances of MUC5B and TTF-1 expressions in resected non-small cell lung cancer. Sci Rep 2015;5:8649. [Crossref] [PubMed]
- Song L, Guan XJ, Chen X, et al. Mesenchymal stem cells reduce cigarette smoke-induced inflammation and airflow obstruction in rats via TGF-β1 signaling. COPD 2014;11:582-90. [Crossref] [PubMed]
- Xu GH, Shen J, Sun P, et al. Anti-inflammatory effects of potato extract on a rat model of cigarette smoke-induced chronic obstructive pulmonary disease. Food Nutr Res 2015;59:28879. [Crossref] [PubMed]
- Terada K, Muro S, Sato S, et al. Impact of gastro-oesophageal reflux disease symptoms on COPD exacerbation. Thorax 2008;63:951-5. [Crossref] [PubMed]
- Wang Q, Wang Y, Zhang Y, et al. The role of uPAR in epithelial-mesenchymal transition in small airway epithelium of patients with chronic obstructive pulmonary disease. Respir Res 2013;14:67. [Crossref] [PubMed]
- Mahmood MQ, Sohal SS, Shukla SD, et al. Epithelial mesenchymal transition in smokers: large versus small airways and relation to airflow obstruction. Int J Chron Obstruct Pulmon Dis 2015;10:1515-24. [Crossref] [PubMed]
- Lee JY, Lee KT, Lee JK, et al. Farnesoid X receptor, overexpressed in pancreatic cancer with lymph node metastasis promotes cell migration and invasion. Br J Cancer 2011;104:1027-37. [Crossref] [PubMed]
- Lin CC, Lee IT, Yang YL, et al. Induction of COX-2/PGE(2)/IL-6 is crucial for cigarette smoke extract-induced airway inflammation: Role of TLR4-dependent NADPH oxidase activation. Free Radic Biol Med 2010;48:240-54. [Crossref] [PubMed]
- Guan B, Li H, Yang Z, et al. Inhibition of farnesoid X receptor controls esophageal cancer cell growth in vitro and in nude mouse xenografts. Cancer 2013;119:1321-9. [Crossref] [PubMed]
- Wu YC, Hsu PK, Su KC, et al. Bile acid aspiration in suspected ventilator-associated pneumonia. Chest 2009;136:118-24. [Crossref] [PubMed]
- Pauwels A, Decraene A, Blondeau K, et al. Bile acids in sputum and increased airway inflammation in patients with cystic fibrosis. Chest 2012;141:1568-74. [Crossref] [PubMed]
- Savarino E, Carbone R, Marabotto E, et al. Gastro-oesophageal reflux and gastric aspiration in idiopathic pulmonary fibrosis patients. Eur Respir J 2013;42:1322-31. [Crossref] [PubMed]
- Nofziger C, Vezzoli V, Dossena S, et al. STAT6 links IL-4/IL-13 stimulation with pendrin expression in asthma and chronic obstructive pulmonary disease. Clin Pharmacol Ther 2011;90:399-405. [Crossref] [PubMed]
- Miotto D, Ruggieri MP, Boschetto P, et al. Interleukin-13 and -4 expression in the central airways of smokers with chronic bronchitis. Eur Respir J 2003;22:602-8. [Crossref] [PubMed]
- Shaykhiev R, Crystal RG. Early events in the pathogenesis of chronic obstructive pulmonary disease. Smoking-induced reprogramming of airway epithelial basal progenitor cells. Ann Am Thorac Soc 2014;11 Suppl 5:S252-8. [Crossref] [PubMed]